Travelling Wave
When something about the physical world changes, the information about that disturbance gradually moves outwards, away from the source, in every direction. As the information travels, it travels in the form of a wave. Sound to our ears, light to our eyes, and electromagnetic radiation to our mobile phones are all transported in the form of waves. A good visual example of the propagation of waves is the waves created on the surface of the water when a stone is dropped into a lake. In this article, we will be learning more about travelling waves.

Describing a Wave
A wave can be described as a disturbance in a medium that travels transferring momentum and energy without any net motion of the medium. A wave in which the positions of maximum and minimum amplitude travel through the medium is known as a travelling wave. To better understand a wave, let us think of the disturbance caused when we jump on a trampoline. When we jump on a trampoline, the downward push that we create at a point on the trampoline slightly moves the material next to it downward too.
When the created disturbance travels outward, the point at which our feet first hit the trampoline recovers moving outward because of the tension force in the trampoline and that moves the surrounding nearby materials outward too. This up and down motion gradually ripples out as it covers more area of the trampoline. And, this disturbance takes the shape of a wave.
Following are a few important points to remember about the wave:
- The high points in the wave are known as crests and the low points in the wave are known as troughs.
- The maximum distance of the disturbance of the wave from the mid-point to either the top of the crest or to the bottom of a trough is known as amplitude.
- The distance between two adjacent crests or two adjacent troughs is known as a wavelength and is denoted by 𝛌.
- The time interval of one complete vibration is known as a time period.
- The number of vibrations the wave undergoes in one second is known as a frequency.
- The relationship between the time period and frequency is given as follows:
- The speed of a wave is given by the equation
Different Types of Waves
Different types of waves exhibit distinct characteristics. These characteristics help us distinguish between wave types. The orientation of particle motion relative to the direction of wave propagation is one way the traveling waves are distinguished. Following are the different types of waves categorized based on the particle motion:
- Pulse Waves – A pulse wave is a wave comprising only one disturbance or only one crest that travels through the transmission medium.
- Continuous Waves – A continuous-wave is a waveform of constant amplitude and frequency.
- Transverse Waves – In a transverse wave, the motion of the particle is perpendicular to the direction of propagation of the wave.
- Longitudinal Waves – Longitudinal waves are the waves in which the motion of the particle is in the same direction as the propagation of the wave.
Although they are different, there is one property common between them and that is the transportation of energy. An object in simple harmonic motion has an energy of
Constructive and Destructive Interference
A phenomenon in which two waves superimpose to form a resultant wave of lower, greater, or the same amplitude is known as interference. Constructive and destructive interference occurs due to the interaction of waves that are correlated with each other either because of the same frequency or because they come from the same source. The interference effects can be observed in all types of waves such as gravity waves and light waves.
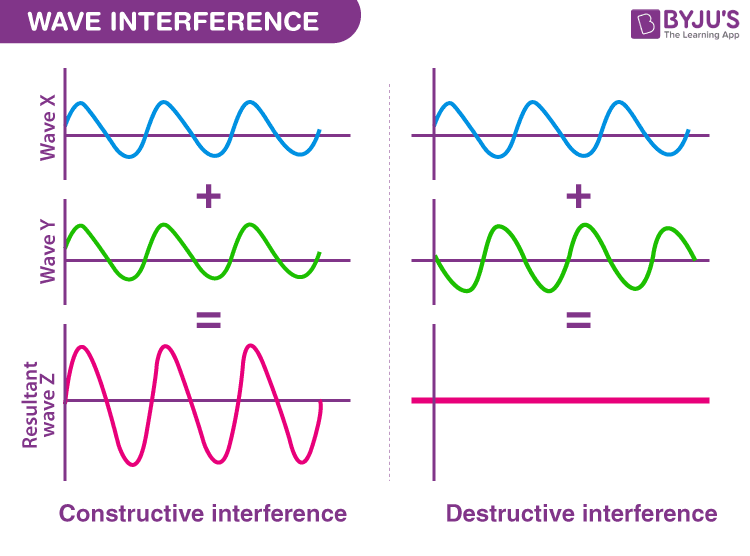
According to the principle of superposition of the waves , when two or more propagating waves of the same type are incidents on the same point, the resultant amplitude is equal to the vector sum of the amplitudes of the individual waves. When a crest of a wave meets a crest of another wave of the same frequency at the same point, then the resultant amplitude is the sum of the individual amplitudes. This type of interference is known as constructive interference. If a crest of a wave meets a trough of another wave, then the resulting amplitude is equal to the difference in the individual amplitudes and this is known as destructive interference.
Stay tuned to BYJU’S to learn more physics concepts with the help of interactive videos.
Watch the video and understand longitudinal and transverse waves in detail.
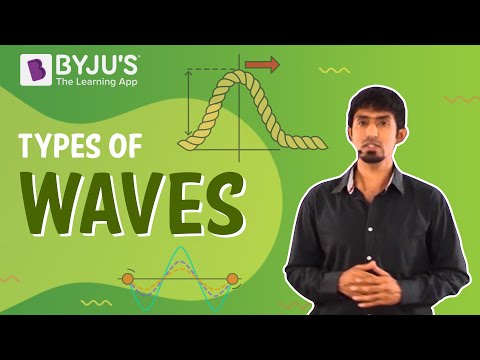
Frequently Asked Questions – FAQs
What is a pulse wave, what are longitudinal waves, what is superposition of waves, what is electromagnetic radiation, what is constructive interference.
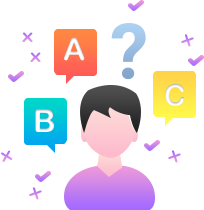
Put your understanding of this concept to test by answering a few MCQs. Click ‘Start Quiz’ to begin!
Select the correct answer and click on the “Finish” button Check your score and answers at the end of the quiz
Visit BYJU’S for all Physics related queries and study materials
Your result is as below
Request OTP on Voice Call
Leave a Comment Cancel reply
Your Mobile number and Email id will not be published. Required fields are marked *
Post My Comment

- Share Share
Register with BYJU'S & Download Free PDFs
Register with byju's & watch live videos.

16.1 Traveling Waves
Learning objectives.
By the end of this section, you will be able to:
- Describe the basic characteristics of wave motion
- Define the terms wavelength, amplitude, period, frequency, and wave speed
- Explain the difference between longitudinal and transverse waves, and give examples of each type
- List the different types of waves
We saw in Oscillations that oscillatory motion is an important type of behavior that can be used to model a wide range of physical phenomena. Oscillatory motion is also important because oscillations can generate waves, which are of fundamental importance in physics. Many of the terms and equations we studied in the chapter on oscillations apply equally well to wave motion ( (Figure) ).
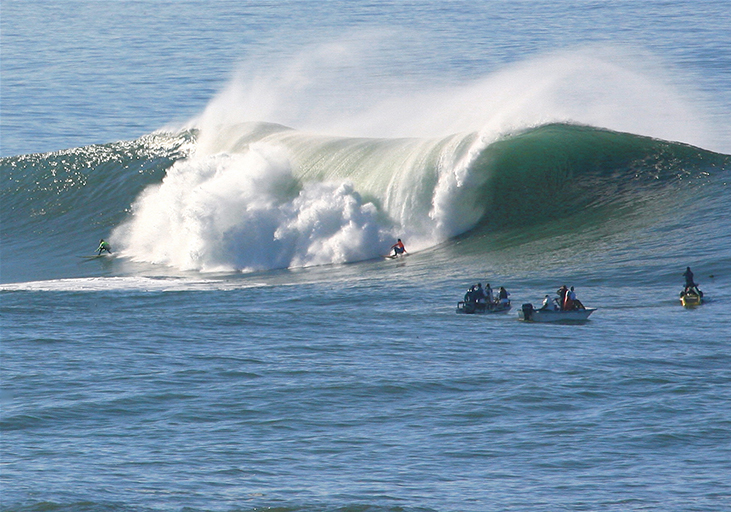
Figure 16.2 From the world of renewable energy sources comes the electric power-generating buoy. Although there are many versions, this one converts the up-and-down motion, as well as side-to-side motion, of the buoy into rotational motion in order to turn an electric generator, which stores the energy in batteries.
Types of Waves
A wave is a disturbance that propagates, or moves from the place it was created. There are three basic types of waves: mechanical waves, electromagnetic waves, and matter waves.
Basic mechanical waves are governed by Newton’s laws and require a medium. A medium is the substance a mechanical waves propagates through, and the medium produces an elastic restoring force when it is deformed. Mechanical waves transfer energy and momentum, without transferring mass. Some examples of mechanical waves are water waves, sound waves, and seismic waves. The medium for water waves is water; for sound waves, the medium is usually air. (Sound waves can travel in other media as well; we will look at that in more detail in Sound .) For surface water waves, the disturbance occurs on the surface of the water, perhaps created by a rock thrown into a pond or by a swimmer splashing the surface repeatedly. For sound waves, the disturbance is a change in air pressure, perhaps created by the oscillating cone inside a speaker or a vibrating tuning fork. In both cases, the disturbance is the oscillation of the molecules of the fluid. In mechanical waves, energy and momentum transfer with the motion of the wave, whereas the mass oscillates around an equilibrium point. (We discuss this in Energy and Power of a Wave .) Earthquakes generate seismic waves from several types of disturbances, including the disturbance of Earth’s surface and pressure disturbances under the surface. Seismic waves travel through the solids and liquids that form Earth. In this chapter, we focus on mechanical waves.
Electromagnetic waves are associated with oscillations in electric and magnetic fields and do not require a medium. Examples include gamma rays, X-rays, ultraviolet waves, visible light, infrared waves, microwaves, and radio waves. Electromagnetic waves can travel through a vacuum at the speed of light, [latex] v=c=2.99792458\,×\,{10}^{8}\,\text{m/s}. [/latex] For example, light from distant stars travels through the vacuum of space and reaches Earth. Electromagnetic waves have some characteristics that are similar to mechanical waves; they are covered in more detail in Electromagnetic Waves in volume 2 of this text.
Matter waves are a central part of the branch of physics known as quantum mechanics. These waves are associated with protons, electrons, neutrons, and other fundamental particles found in nature. The theory that all types of matter have wave-like properties was first proposed by Louis de Broglie in 1924. Matter waves are discussed in Photons and Matter Waves in the third volume of this text.
Mechanical Waves
Mechanical waves exhibit characteristics common to all waves, such as amplitude, wavelength, period, frequency, and energy. All wave characteristics can be described by a small set of underlying principles.
The simplest mechanical waves repeat themselves for several cycles and are associated with simple harmonic motion. These simple harmonic waves can be modeled using some combination of sine and cosine functions. For example, consider the simplified surface water wave that moves across the surface of water as illustrated in (Figure) . Unlike complex ocean waves, in surface water waves, the medium, in this case water, moves vertically, oscillating up and down, whereas the disturbance of the wave moves horizontally through the medium. In (Figure) , the waves causes a seagull to move up and down in simple harmonic motion as the wave crests and troughs (peaks and valleys) pass under the bird. The crest is the highest point of the wave, and the trough is the lowest part of the wave. The time for one complete oscillation of the up-and-down motion is the wave’s period T . The wave’s frequency is the number of waves that pass through a point per unit time and is equal to [latex] f=1\text{/}T. [/latex] The period can be expressed using any convenient unit of time but is usually measured in seconds; frequency is usually measured in hertz (Hz), where [latex] 1\,{\text{Hz}=1\,\text{s}}^{-1}. [/latex]
The length of the wave is called the wavelength and is represented by the Greek letter lambda [latex] (\lambda ) [/latex], which is measured in any convenient unit of length, such as a centimeter or meter. The wavelength can be measured between any two similar points along the medium that have the same height and the same slope. In (Figure) , the wavelength is shown measured between two crests. As stated above, the period of the wave is equal to the time for one oscillation, but it is also equal to the time for one wavelength to pass through a point along the wave’s path.
The amplitude of the wave ( A ) is a measure of the maximum displacement of the medium from its equilibrium position. In the figure, the equilibrium position is indicated by the dotted line, which is the height of the water if there were no waves moving through it. In this case, the wave is symmetrical, the crest of the wave is a distance [latex] \text{+}A [/latex] above the equilibrium position, and the trough is a distance [latex] \text{−}A [/latex] below the equilibrium position. The units for the amplitude can be centimeters or meters, or any convenient unit of distance.
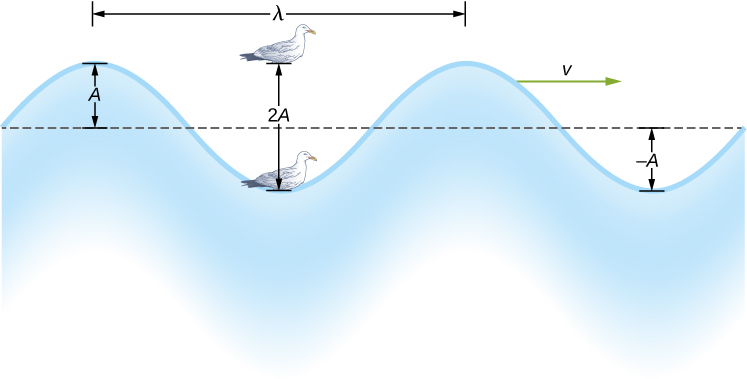
Figure 16.3 An idealized surface water wave passes under a seagull that bobs up and down in simple harmonic motion. The wave has a wavelength [latex] \lambda [/latex], which is the distance between adjacent identical parts of the wave. The amplitude A of the wave is the maximum displacement of the wave from the equilibrium position, which is indicated by the dotted line. In this example, the medium moves up and down, whereas the disturbance of the surface propagates parallel to the surface at a speed v.
The water wave in the figure moves through the medium with a propagation velocity [latex] \overset{\to }{v}. [/latex] The magnitude of the wave velocity is the distance the wave travels in a given time, which is one wavelength in the time of one period, and the wave speed is the magnitude of wave velocity. In equation form, this is
This fundamental relationship holds for all types of waves. For water waves, v is the speed of a surface wave; for sound, v is the speed of sound; and for visible light, v is the speed of light.
Transverse and Longitudinal Waves
We have seen that a simple mechanical wave consists of a periodic disturbance that propagates from one place to another through a medium. In (Figure) (a), the wave propagates in the horizontal direction, whereas the medium is disturbed in the vertical direction. Such a wave is called a transverse wave . In a transverse wave, the wave may propagate in any direction, but the disturbance of the medium is perpendicular to the direction of propagation. In contrast, in a longitudinal wave or compressional wave, the disturbance is parallel to the direction of propagation. (Figure) (b) shows an example of a longitudinal wave. The size of the disturbance is its amplitude A and is completely independent of the speed of propagation v .
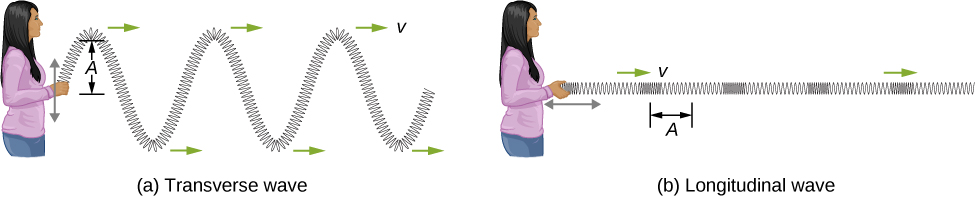
Figure 16.4 (a) In a transverse wave, the medium oscillates perpendicular to the wave velocity. Here, the spring moves vertically up and down, while the wave propagates horizontally to the right. (b) In a longitudinal wave, the medium oscillates parallel to the propagation of the wave. In this case, the spring oscillates back and forth, while the wave propagates to the right.
A simple graphical representation of a section of the spring shown in (Figure) (b) is shown in (Figure) . (Figure) (a) shows the equilibrium position of the spring before any waves move down it. A point on the spring is marked with a blue dot. (Figure) (b) through (g) show snapshots of the spring taken one-quarter of a period apart, sometime after the end of` the spring is oscillated back and forth in the x -direction at a constant frequency. The disturbance of the wave is seen as the compressions and the expansions of the spring. Note that the blue dot oscillates around its equilibrium position a distance A , as the longitudinal wave moves in the positive x -direction with a constant speed. The distance A is the amplitude of the wave. The y -position of the dot does not change as the wave moves through the spring. The wavelength of the wave is measured in part (d). The wavelength depends on the speed of the wave and the frequency of the driving force.
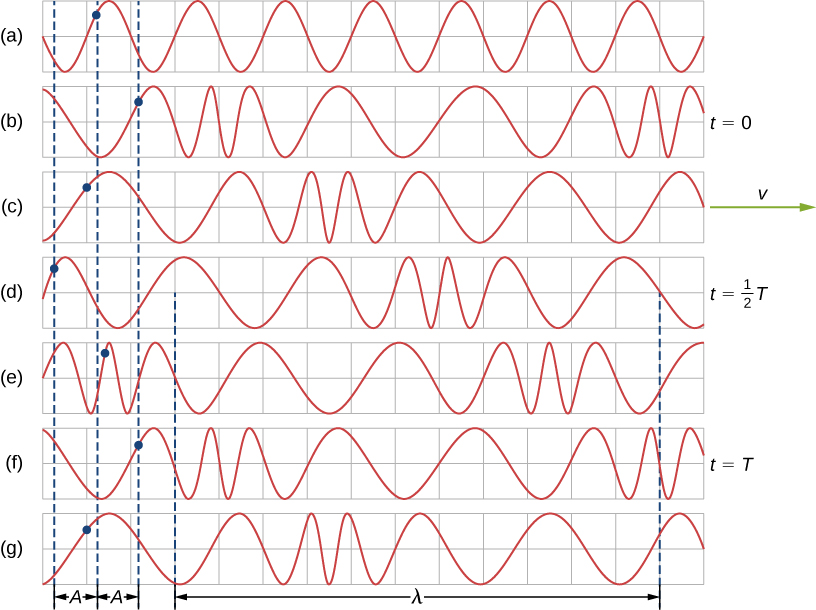
Figure 16.5 (a) This is a simple, graphical representation of a section of the stretched spring shown in (Figure)(b), representing the spring’s equilibrium position before any waves are induced on the spring. A point on the spring is marked by a blue dot. (b–g) Longitudinal waves are created by oscillating the end of the spring (not shown) back and forth along the x-axis. The longitudinal wave, with a wavelength [latex] \lambda [/latex], moves along the spring in the +x-direction with a wave speed v. For convenience, the wavelength is measured in (d). Note that the point on the spring that was marked with the blue dot moves back and forth a distance A from the equilibrium position, oscillating around the equilibrium position of the point.
Waves may be transverse, longitudinal, or a combination of the two. Examples of transverse waves are the waves on stringed instruments or surface waves on water, such as ripples moving on a pond. Sound waves in air and water are longitudinal. With sound waves, the disturbances are periodic variations in pressure that are transmitted in fluids. Fluids do not have appreciable shear strength, and for this reason, the sound waves in them are longitudinal waves. Sound in solids can have both longitudinal and transverse components, such as those in a seismic wave. Earthquakes generate seismic waves under Earth’s surface with both longitudinal and transverse components (called compressional or P-waves and shear or S-waves, respectively). The components of seismic waves have important individual characteristics—they propagate at different speeds, for example. Earthquakes also have surface waves that are similar to surface waves on water. Ocean waves also have both transverse and longitudinal components.
Wave on a String
A student takes a 30.00-m-long string and attaches one end to the wall in the physics lab. The student then holds the free end of the rope, keeping the tension constant in the rope. The student then begins to send waves down the string by moving the end of the string up and down with a frequency of 2.00 Hz. The maximum displacement of the end of the string is 20.00 cm. The first wave hits the lab wall 6.00 s after it was created. (a) What is the speed of the wave? (b) What is the period of the wave? (c) What is the wavelength of the wave?
- The speed of the wave can be derived by dividing the distance traveled by the time.
- The period of the wave is the inverse of the frequency of the driving force.
- The wavelength can be found from the speed and the period [latex] v=\lambda \text{/}T. [/latex]
- The first wave traveled 30.00 m in 6.00 s: [latex] v=\frac{30.00\,\text{m}}{6.00\,\text{s}}=5.00\frac{\text{m}}{\text{s}}. [/latex]
- The period is equal to the inverse of the frequency: [latex] T=\frac{1}{f}=\frac{1}{2.00\,{\text{s}}^{-1}}=0.50\,\text{s}. [/latex]
- The wavelength is equal to the velocity times the period: [latex] \lambda =vT=5.00\frac{\text{m}}{\text{s}}(0.50\,\text{s})=2.50\,\text{m}. [/latex]
Significance
The frequency of the wave produced by an oscillating driving force is equal to the frequency of the driving force.
Check Your Understanding
When a guitar string is plucked, the guitar string oscillates as a result of waves moving through the string. The vibrations of the string cause the air molecules to oscillate, forming sound waves. The frequency of the sound waves is equal to the frequency of the vibrating string. Is the wavelength of the sound wave always equal to the wavelength of the waves on the string?
The wavelength of the waves depends on the frequency and the velocity of the wave. The frequency of the sound wave is equal to the frequency of the wave on the string. The wavelengths of the sound waves and the waves on the string are equal only if the velocities of the waves are the same, which is not always the case. If the speed of the sound wave is different from the speed of the wave on the string, the wavelengths are different. This velocity of sound waves will be discussed in Sound .
Characteristics of a Wave
A transverse mechanical wave propagates in the positive x -direction through a spring (as shown in (Figure) (a)) with a constant wave speed, and the medium oscillates between [latex] \text{+}A [/latex] and [latex] \text{−}A [/latex] around an equilibrium position. The graph in (Figure) shows the height of the spring ( y ) versus the position ( x ), where the x -axis points in the direction of propagation. The figure shows the height of the spring versus the x -position at [latex] t=0.00\,\text{s} [/latex] as a dotted line and the wave at [latex] t=3.00\,\text{s} [/latex] as a solid line. (a) Determine the wavelength and amplitude of the wave. (b) Find the propagation velocity of the wave. (c) Calculate the period and frequency of the wave.
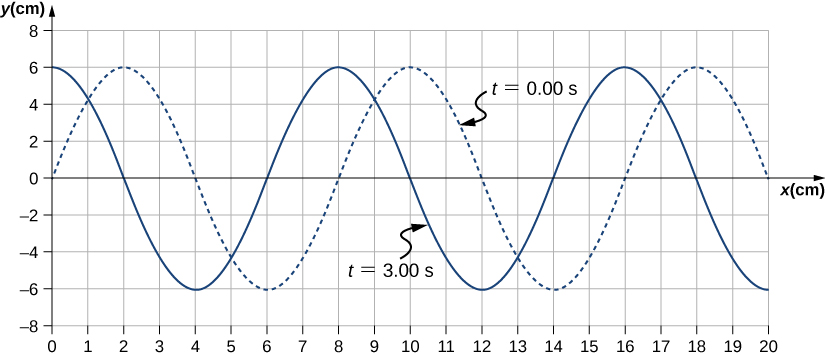
Figure 16.6 A transverse wave shown at two instants of time.
- The amplitude and wavelength can be determined from the graph.
- Since the velocity is constant, the velocity of the wave can be found by dividing the distance traveled by the wave by the time it took the wave to travel the distance.
- The period can be found from [latex] v=\frac{\lambda }{T} [/latex] and the frequency from [latex] f=\frac{1}{T}. [/latex]
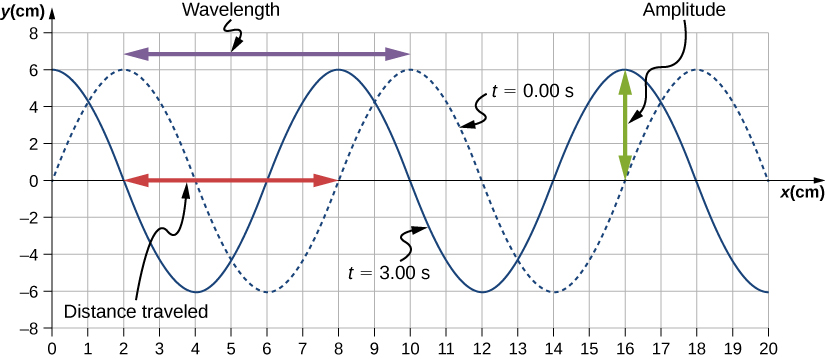
Figure 16.7 Characteristics of the wave marked on a graph of its displacement.
- The distance the wave traveled from time [latex] t=0.00\,\text{s} [/latex] to time [latex] t=3.00\,\text{s} [/latex] can be seen in the graph. Consider the red arrow, which shows the distance the crest has moved in 3 s. The distance is [latex] 8.00\,\text{cm}-2.00\,\text{cm}=6.00\,\text{cm}. [/latex] The velocity is [latex] v=\frac{\text{Δ}x}{\text{Δ}t}=\frac{8.00\,\text{cm}-2.00\,\text{cm}}{3.00\,\text{s}-0.00\,\text{s}}=2.00\,\text{cm/s}. [/latex]
- The period is [latex] T=\frac{\lambda }{v}=\frac{8.00\,\text{cm}}{2.00\,\text{cm/s}}=4.00\,\text{s} [/latex] and the frequency is [latex] f=\frac{1}{T}=\frac{1}{4.00\,\text{s}}=0.25\,\text{Hz}. [/latex]
Note that the wavelength can be found using any two successive identical points that repeat, having the same height and slope. You should choose two points that are most convenient. The displacement can also be found using any convenient point.
The propagation velocity of a transverse or longitudinal mechanical wave may be constant as the wave disturbance moves through the medium. Consider a transverse mechanical wave: Is the velocity of the medium also constant?
- A wave is a disturbance that moves from the point of origin with a wave velocity v .
- A wave has a wavelength [latex] \lambda [/latex], which is the distance between adjacent identical parts of the wave. Wave velocity and wavelength are related to the wave’s frequency and period by [latex] v=\frac{\lambda }{T}=\lambda f. [/latex]
- Mechanical waves are disturbances that move through a medium and are governed by Newton’s laws.
- Electromagnetic waves are disturbances in the electric and magnetic fields, and do not require a medium.
- Matter waves are a central part of quantum mechanics and are associated with protons, electrons, neutrons, and other fundamental particles found in nature.
- A transverse wave has a disturbance perpendicular to the wave’s direction of propagation, whereas a longitudinal wave has a disturbance parallel to its direction of propagation.
Conceptual Questions
Give one example of a transverse wave and one example of a longitudinal wave, being careful to note the relative directions of the disturbance and wave propagation in each.
A sinusoidal transverse wave has a wavelength of 2.80 m. It takes 0.10 s for a portion of the string at a position x to move from a maximum position of [latex] y=0.03\,\text{m} [/latex] to the equilibrium position [latex] y=0. [/latex] What are the period, frequency, and wave speed of the wave?
What is the difference between propagation speed and the frequency of a mechanical wave? Does one or both affect wavelength? If so, how?
Propagation speed is the speed of the wave propagating through the medium. If the wave speed is constant, the speed can be found by [latex] v=\frac{\lambda }{T}=\lambda f. [/latex] The frequency is the number of wave that pass a point per unit time. The wavelength is directly proportional to the wave speed and inversely proportional to the frequency.
Consider a stretched spring, such as a slinky. The stretched spring can support longitudinal waves and transverse waves. How can you produce transverse waves on the spring? How can you produce longitudinal waves on the spring?
Consider a wave produced on a stretched spring by holding one end and shaking it up and down. Does the wavelength depend on the distance you move your hand up and down?
No, the distance you move your hand up and down will determine the amplitude of the wave. The wavelength will depend on the frequency you move your hand up and down, and the speed of the wave through the spring.
A sinusoidal, transverse wave is produced on a stretched spring, having a period T . Each section of the spring moves perpendicular to the direction of propagation of the wave, in simple harmonic motion with an amplitude A . Does each section oscillate with the same period as the wave or a different period? If the amplitude of the transverse wave were doubled but the period stays the same, would your answer be the same?
An electromagnetic wave, such as light, does not require a medium. Can you think of an example that would support this claim?
Storms in the South Pacific can create waves that travel all the way to the California coast, 12,000 km away. How long does it take them to travel this distance if they travel at 15.0 m/s?
Waves on a swimming pool propagate at 0.75 m/s. You splash the water at one end of the pool and observe the wave go to the opposite end, reflect, and return in 30.00 s. How far away is the other end of the pool?
[latex] 2d=vt⇒d=11.25\,\text{m} [/latex]
Wind gusts create ripples on the ocean that have a wavelength of 5.00 cm and propagate at 2.00 m/s. What is their frequency?
How many times a minute does a boat bob up and down on ocean waves that have a wavelength of 40.0 m and a propagation speed of 5.00 m/s?
Scouts at a camp shake the rope bridge they have just crossed and observe the wave crests to be 8.00 m apart. If they shake the bridge twice per second, what is the propagation speed of the waves?
What is the wavelength of the waves you create in a swimming pool if you splash your hand at a rate of 2.00 Hz and the waves propagate at a wave speed of 0.800 m/s?
[latex] v=f\lambda ⇒\lambda =0.400\,\text{m} [/latex]
What is the wavelength of an earthquake that shakes you with a frequency of 10.0 Hz and gets to another city 84.0 km away in 12.0 s?
Radio waves transmitted through empty space at the speed of light [latex] (v=c=3.00\,×\,{10}^{8}\,\text{m/s}) [/latex] by the Voyager spacecraft have a wavelength of 0.120 m. What is their frequency?
Your ear is capable of differentiating sounds that arrive at each ear just 0.34 ms apart, which is useful in determining where low frequency sound is originating from. (a) Suppose a low-frequency sound source is placed to the right of a person, whose ears are approximately 18 cm apart, and the speed of sound generated is 340 m/s. How long is the interval between when the sound arrives at the right ear and the sound arrives at the left ear? (b) Assume the same person was scuba diving and a low-frequency sound source was to the right of the scuba diver. How long is the interval between when the sound arrives at the right ear and the sound arrives at the left ear, if the speed of sound in water is 1500 m/s? (c) What is significant about the time interval of the two situations?
(a) Seismographs measure the arrival times of earthquakes with a precision of 0.100 s. To get the distance to the epicenter of the quake, geologists compare the arrival times of S- and P-waves, which travel at different speeds. If S- and P-waves travel at 4.00 and 7.20 km/s, respectively, in the region considered, how precisely can the distance to the source of the earthquake be determined? (b) Seismic waves from underground detonations of nuclear bombs can be used to locate the test site and detect violations of test bans. Discuss whether your answer to (a) implies a serious limit to such detection. (Note also that the uncertainty is greater if there is an uncertainty in the propagation speeds of the S- and P-waves.)
a. The P-waves outrun the S-waves by a speed of [latex] v=3.20\,\text{km/s;} [/latex] therefore, [latex] \text{Δ}d=0.320\,\text{km}. [/latex] b. Since the uncertainty in the distance is less than a kilometer, our answer to part (a) does not seem to limit the detection of nuclear bomb detonations. However, if the velocities are uncertain, then the uncertainty in the distance would increase and could then make it difficult to identify the source of the seismic waves.
A Girl Scout is taking a 10.00-km hike to earn a merit badge. While on the hike, she sees a cliff some distance away. She wishes to estimate the time required to walk to the cliff. She knows that the speed of sound is approximately 343 meters per second. She yells and finds that the echo returns after approximately 2.00 seconds. If she can hike 1.00 km in 10 minutes, how long would it take her to reach the cliff?
A quality assurance engineer at a frying pan company is asked to qualify a new line of nonstick-coated frying pans. The coating needs to be 1.00 mm thick. One method to test the thickness is for the engineer to pick a percentage of the pans manufactured, strip off the coating, and measure the thickness using a micrometer. This method is a destructive testing method. Instead, the engineer decides that every frying pan will be tested using a nondestructive method. An ultrasonic transducer is used that produces sound waves with a frequency of [latex] f=25\,\text{kHz}. [/latex] The sound waves are sent through the coating and are reflected by the interface between the coating and the metal pan, and the time is recorded. The wavelength of the ultrasonic waves in the coating is 0.076 m. What should be the time recorded if the coating is the correct thickness (1.00 mm)?
- OpenStax University Physics. Authored by : OpenStax CNX. Located at : https://cnx.org/contents/[email protected]:Gofkr9Oy@15 . License : CC BY: Attribution . License Terms : Download for free at http://cnx.org/contents/[email protected]
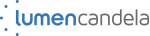
Privacy Policy
Forgot password? New user? Sign up
Existing user? Log in
Understanding Basic Traveling Waves
Already have an account? Log in here.
After learning the basics of periodic motion, it's time to take up the study of oscillations to the next level. By the next level, I mean the dependence of the motion of the considered particle on not just the time variable, but also on the distance variable. Let's dive right into the topic by first differentiating waves.
Types of Waves
What do we mean by travelling waves, equation representing a travelling wave.
We all have heard about various kinds of of waves traveling around us. Starting from the most familiar waves of light and sound, to the complex matter waves, all of them follow a common feature, i.e. oscillation of energy.
That is what a wave is.
A wave is just the phenomenon of oscillation of energy, using various properties of a medium such as physical, electro-magnetic, etc. For example, sound waves and light waves are both the carriers of energy, but a sound wave propagates through pressure variations, whereas a light wave travels by making use of electro-magnetic phenomena, which we'll discuss shortly.
Differentiating waves on alignment of propagation with respect to oscillation:
- Longitudinal waves: The type of waves that move in such a way that the oscillation of energy is along the direction of motion of the wave is defined as a longitudinal wave . Such kind of wave can be viewed by imagining two friends, who are walking forward, one ahead of the other, such that they continue tossing a ball between each other. In this, their motion can be seen as the motion of a wave and the ball acts as the packet of energy. These waves are also known as pressure waves .
- Transverse waves: The type of waves that move in such a way that the oscillation of energy is perpendicular to the direction of the motion of the wave is defined as transverse wave. Such kind of wave can be pictured by imagining the same two friends, walking forward, but one beside another, such that they continue tossing a ball between each other. In this, their motion can be seen as the motion of a wave and the ball acts as the packet of energy.
Imagine stretching a string and fixing both its ends on two points. Now grab the mid point of the string and pull it down, before letting it go. Chances are that you'll see the mid point of the string oscillating with an amplitude, with the end points fixed at their respective positions. These kind of waves are what we call the standing waves .
Now, for the next experiment, get into a hall with your friend and call out to him. If you shout loud enough and your friend hears well, chances are that he'll hear your call. Your voice reached him by the motion of sound waves, i.e. travelling waves . Had the sound waves been stationary, your voice would have never reached him.
We all have read about the basic mathematical equation that governs the trajectory or the position of a particle in SHM, or in other words, a particle belonging to a stationary wave. It is given by \(y(t)=a\sin \omega t \), where \(a\) represents the maximum displacement of the particle from the mean position ( amplitude ), and \(\omega\) represents the angular frequency for the SHM.
Now, since a travelling wave also moves forward while changing with time, a similar equation in case of a travelling wave must definitely include a function of both the direction of propagation (let it be \(z\)) and time. So, we get: \(y\left( z,t \right) =a\sin [z,t]\), where the symbols have their usual meanings.
To find this function that will explain the oscillation of the particle, we use the basic property of linearity of the function, i.e. the function enclosed inside the \(\sin\) block must be a linear function of \(z\) and \(t,\) or else the oscillating graph would lose its shape and the wave would compress or stretch out in different locations.
So, let that be given by \(\Phi \left( z,t \right)=\alpha z+\beta t\), but since we assume the wave to be travelling towards \(z=+\infty\), \(\alpha\) and \(\beta\) must have opposite signs. Therefore,
\[\Phi \left( z,t \right)=|\alpha |z-|\beta |t.\]
Also, we already know that this function must have the dimensions of radians , so \(\beta\) has the dimensions of \(T^{-1}\) and \(\alpha\) has the dimensions of \(L^{-1}\). As it turns out, \(\alpha\) is given by a constant \(k\) that is known as the wave-number for the wave, and equals \(\frac{2\pi}{\lambda}\), where \(\lambda\) is the wavelength of the wave. On the other hand, \(\beta\) is our age old friend \(\omega\) which equals \(2\pi\nu\), where \(\nu\) is the frequency.
So, we end up with: \(y\left( z,t \right) =a\sin ({k z-\omega t}). \)
Note: If the wave travels towards \(-\infty\), the function would change to \(y\left( z,t \right) =a\sin ({k z+\omega t}) .\)
Problem Loading...
Note Loading...
Set Loading...
If you're seeing this message, it means we're having trouble loading external resources on our website.
If you're behind a web filter, please make sure that the domains *.kastatic.org and *.kasandbox.org are unblocked.
To log in and use all the features of Khan Academy, please enable JavaScript in your browser.
Middle school physics - NGSS
Course: middle school physics - ngss > unit 4, wave properties.
- Understand: wave properties
- Apply: wave properties
Key points:
- A wave is a repeating disturbance that travels through matter or space transferring only energy.
- Below is a model of a wave.
- A wave’s crest is its highest point, and its trough is its lowest point.
- A wave’s amplitude is the maximum distance (positive or negative) a wave reaches from its rest position.
- Wavelength is the distance between the same spot on two sections of a wave.
- A wave’s frequency can be measured by how many crests (or how many troughs) pass a location in a certain amount of time.
- A wave with a larger frequency has more energy. If a wave’s frequency doubles, its energy also doubles.
- A wave’s energy is proportional to the square of its amplitude. So, if a wave’s amplitude doubles, its energy increases by four times, because 2 2 = 4 .
Want to join the conversation?
- Upvote Button navigates to signup page
- Downvote Button navigates to signup page
- Flag Button navigates to signup page

13.2 Wave Properties: Speed, Amplitude, Frequency, and Period
Section learning objectives.
By the end of this section, you will be able to do the following:
- Define amplitude, frequency, period, wavelength, and velocity of a wave
- Relate wave frequency, period, wavelength, and velocity
- Solve problems involving wave properties
Teacher Support
The learning objectives in this section will help your students master the following standards:
- (B) investigate and analyze the characteristics of waves, including velocity, frequency, amplitude, and wavelength, and calculate using the relationship between wave speed, frequency, and wavelength;
- (D) investigate the behaviors of waves, including reflection, refraction, diffraction, interference, resonance, and the Doppler effect.
Section Key Terms
[BL] [OL] [AL] Review amplitude, period, and frequency for simple harmonic motion.
Wave Variables
In the chapter on motion in two dimensions, we defined the following variables to describe harmonic motion:
- Amplitude—maximum displacement from the equilibrium position of an object oscillating around such equilibrium position
- Frequency—number of events per unit of time
- Period—time it takes to complete one oscillation
For waves, these variables have the same basic meaning. However, it is helpful to word the definitions in a more specific way that applies directly to waves:
- Amplitude—distance between the resting position and the maximum displacement of the wave
- Frequency—number of waves passing by a specific point per second
- Period—time it takes for one wave cycle to complete
In addition to amplitude, frequency, and period, their wavelength and wave velocity also characterize waves. The wavelength λ λ is the distance between adjacent identical parts of a wave, parallel to the direction of propagation. The wave velocity v w v w is the speed at which the disturbance moves.
Tips For Success
Wave velocity is sometimes also called the propagation velocity or propagation speed because the disturbance propagates from one location to another.
Consider the periodic water wave in Figure 13.7 . Its wavelength is the distance from crest to crest or from trough to trough. The wavelength can also be thought of as the distance a wave has traveled after one complete cycle—or one period. The time for one complete up-and-down motion is the simple water wave’s period T . In the figure, the wave itself moves to the right with a wave velocity v w . Its amplitude X is the distance between the resting position and the maximum displacement—either the crest or the trough—of the wave. It is important to note that this movement of the wave is actually the disturbance moving to the right, not the water itself; otherwise, the bird would move to the right. Instead, the seagull bobs up and down in place as waves pass underneath, traveling a total distance of 2 X in one cycle. However, as mentioned in the text feature on surfing, actual ocean waves are more complex than this simplified example.
Watch Physics
Amplitude, period, frequency, and wavelength of periodic waves.
This video is a continuation of the video “Introduction to Waves” from the "Types of Waves" section. It discusses the properties of a periodic wave: amplitude, period, frequency, wavelength, and wave velocity.
The crest of a wave is sometimes also called the peak .
The Relationship between Wave Frequency, Period, Wavelength, and Velocity
Since wave frequency is the number of waves per second, and the period is essentially the number of seconds per wave, the relationship between frequency and period is
just as in the case of harmonic motion of an object. We can see from this relationship that a higher frequency means a shorter period. Recall that the unit for frequency is hertz (Hz), and that 1 Hz is one cycle—or one wave—per second.
The speed of propagation v w is the distance the wave travels in a given time, which is one wavelength in a time of one period. In equation form, it is written as
From this relationship, we see that in a medium where v w is constant, the higher the frequency, the smaller the wavelength. See Figure 13.8 .
[BL] For sound, a higher frequency corresponds to a higher pitch while a lower frequency corresponds to a lower pitch. Amplitude corresponds to the loudness of the sound.
[BL] [OL] Since sound at all frequencies has the same speed in air, a change in frequency means a change in wavelength.
[Figure Support] The same speaker is capable of reproducing both high- and low-frequency sounds. However, high frequencies have shorter wavelengths and are hence best reproduced by a speaker with a small, hard, and tight cone (tweeter), whereas lower frequencies are best reproduced by a large and soft cone (woofer).
These fundamental relationships hold true for all types of waves. As an example, for water waves, v w is the speed of a surface wave; for sound, v w is the speed of sound; and for visible light, v w is the speed of light. The amplitude X is completely independent of the speed of propagation v w and depends only on the amount of energy in the wave.
Waves in a Bowl
In this lab, you will take measurements to determine how the amplitude and the period of waves are affected by the transfer of energy from a cork dropped into the water. The cork initially has some potential energy when it is held above the water—the greater the height, the higher the potential energy. When it is dropped, such potential energy is converted to kinetic energy as the cork falls. When the cork hits the water, that energy travels through the water in waves.
- Large bowl or basin
- Cork (or ping pong ball)
- Measuring tape
Instructions
- Fill a large bowl or basin with water and wait for the water to settle so there are no ripples.
- Gently drop a cork into the middle of the bowl.
- Estimate the wavelength and the period of oscillation of the water wave that propagates away from the cork. You can estimate the period by counting the number of ripples from the center to the edge of the bowl while your partner times it. This information, combined with the bowl measurement, will give you the wavelength when the correct formula is used.
- Remove the cork from the bowl and wait for the water to settle again.
- Gently drop the cork at a height that is different from the first drop.
- Repeat Steps 3 to 5 to collect a second and third set of data, dropping the cork from different heights and recording the resulting wavelengths and periods.
- Interpret your results.
- No, only the amplitude is affected.
- Yes, the wavelength is affected.
Students can measure the bowl beforehand to help them make a better estimation of the wavelength.
Links To Physics
Geology: physics of seismic waves.
Geologists rely heavily on physics to study earthquakes since earthquakes involve several types of wave disturbances, including disturbance of Earth’s surface and pressure disturbances under the surface. Surface earthquake waves are similar to surface waves on water. The waves under Earth’s surface have both longitudinal and transverse components. The longitudinal waves in an earthquake are called pressure waves (P-waves) and the transverse waves are called shear waves (S-waves). These two types of waves propagate at different speeds, and the speed at which they travel depends on the rigidity of the medium through which they are traveling. During earthquakes, the speed of P-waves in granite is significantly higher than the speed of S-waves. Both components of earthquakes travel more slowly in less rigid materials, such as sediments. P-waves have speeds of 4 to 7 km/s, and S-waves have speeds of 2 to 5 km/s, but both are faster in more rigid materials. The P-wave gets progressively farther ahead of the S-wave as they travel through Earth’s crust. For that reason, the time difference between the P- and S-waves is used to determine the distance to their source, the epicenter of the earthquake.
We know from seismic waves produced by earthquakes that parts of the interior of Earth are liquid. Shear or transverse waves cannot travel through a liquid and are not transmitted through Earth’s core. In contrast, compression or longitudinal waves can pass through a liquid and they do go through the core.
All waves carry energy, and the energy of earthquake waves is easy to observe based on the amount of damage left behind after the ground has stopped moving. Earthquakes can shake whole cities to the ground, performing the work of thousands of wrecking balls. The amount of energy in a wave is related to its amplitude. Large-amplitude earthquakes produce large ground displacements and greater damage. As earthquake waves spread out, their amplitude decreases, so there is less damage the farther they get from the source.
Grasp Check
What is the relationship between the propagation speed, frequency, and wavelength of the S-waves in an earthquake?
- The relationship between the propagation speed, frequency, and wavelength is v w = f λ . v w = f λ .
- The relationship between the propagation speed, frequency, and wavelength is v w = λ f . v w = λ f .
Virtual Physics
Wave on a string.
In this animation, watch how a string vibrates in slow motion by choosing the Slow Motion setting. Select the No End and Manual options, and wiggle the end of the string to make waves yourself. Then switch to the Oscillate setting to generate waves automatically. Adjust the frequency and the amplitude of the oscillations to see what happens. Then experiment with adjusting the damping and the tension.
Which of the settings—amplitude, frequency, damping, or tension—changes the amplitude of the wave as it propagates? What does it do to the amplitude?
- Frequency; it decreases the amplitude of the wave as it propagates.
- Frequency; it increases the amplitude of the wave as it propagates.
- Damping; it decreases the amplitude of the wave as it propagates.
- Damping; it increases the amplitude of the wave as it propagates.
Solving Wave Problems
Worked example, calculate the velocity of wave propagation: gull in the ocean.
Calculate the wave velocity of the ocean wave in the previous figure if the distance between wave crests is 10.0 m and the time for a seagull to bob up and down is 5.00 s.
The values for the wavelength ( λ = 10.0 m ) ( λ = 10.0 m ) and the period ( T = 5.00 s) ( T = 5.00 s) are given and we are asked to find v w v w Therefore, we can use v w = λ T v w = λ T to find the wave velocity.
Enter the known values into v w = λ T v w = λ T
This slow speed seems reasonable for an ocean wave. Note that in the figure, the wave moves to the right at this speed, which is different from the varying speed at which the seagull bobs up and down.
Calculate the Period and the Wave Velocity of a Toy Spring
The woman in Figure 13.3 creates two waves every second by shaking the toy spring up and down. (a)What is the period of each wave? (b) If each wave travels 0.9 meters after one complete wave cycle, what is the velocity of wave propagation?
Strategy FOR (A)
To find the period, we solve for T = 1 f T = 1 f , given the value of the frequency ( f = 2 s − 1 ). ( f = 2 s − 1 ).
Enter the known value into T = 1 f T = 1 f
Strategy FOR (B)
Since one definition of wavelength is the distance a wave has traveled after one complete cycle—or one period—the values for the wavelength ( λ = 0.9 m ) ( λ = 0.9 m ) as well as the frequency are given. Therefore, we can use v w = f λ v w = f λ to find the wave velocity.
Enter the known values into v w = f λ v w = f λ
v w = f λ = ( 2 s −1 )(0 .9 m) = 1 .8 m/s . v w = f λ = ( 2 s −1 )(0 .9 m) = 1 .8 m/s .
We could have also used the equation v w = λ T v w = λ T to solve for the wave velocity since we already know the value of the period ( T = 0.5 s) ( T = 0.5 s) from our calculation in part (a), and we would come up with the same answer.
Practice Problems
The frequency of a wave is 10 Hz. What is its period?
- The period of the wave is 100 s.
- The period of the wave is 10 s.
- The period of the wave is 0.01 s.
- The period of the wave is 0.1 s.
What is the velocity of a wave whose wavelength is 2 m and whose frequency is 5 Hz?
Check Your Understanding
Use these questions to assess students’ achievement of the section’s Learning Objectives. If students are struggling with a specific objective, these questions will help identify such objective and direct them to the relevant content.
What is the amplitude of a wave?
- A quarter of the total height of the wave
- Half of the total height of the wave
- Two times the total height of the wave
- Four times the total height of the wave
- The wavelength is the distance between adjacent identical parts of a wave, parallel to the direction of propagation.
- The wavelength is the distance between adjacent identical parts of a wave, perpendicular to the direction of propagation.
- The wavelength is the distance between a crest and the adjacent trough of a wave, parallel to the direction of propagation.
- The wavelength is the distance between a crest and the adjacent trough of a wave, perpendicular to the direction of propagation.
- f = ( 1 T ) 2
- f = ( T ) 2
When is the wavelength directly proportional to the period of a wave?
- When the velocity of the wave is halved
- When the velocity of the wave is constant
- When the velocity of the wave is doubled
- When the velocity of the wave is tripled
As an Amazon Associate we earn from qualifying purchases.
This book may not be used in the training of large language models or otherwise be ingested into large language models or generative AI offerings without OpenStax's permission.
Want to cite, share, or modify this book? This book uses the Creative Commons Attribution License and you must attribute Texas Education Agency (TEA). The original material is available at: https://www.texasgateway.org/book/tea-physics . Changes were made to the original material, including updates to art, structure, and other content updates.
Access for free at https://openstax.org/books/physics/pages/1-introduction
- Authors: Paul Peter Urone, Roger Hinrichs
- Publisher/website: OpenStax
- Book title: Physics
- Publication date: Mar 26, 2020
- Location: Houston, Texas
- Book URL: https://openstax.org/books/physics/pages/1-introduction
- Section URL: https://openstax.org/books/physics/pages/13-2-wave-properties-speed-amplitude-frequency-and-period
© Jan 19, 2024 Texas Education Agency (TEA). The OpenStax name, OpenStax logo, OpenStax book covers, OpenStax CNX name, and OpenStax CNX logo are not subject to the Creative Commons license and may not be reproduced without the prior and express written consent of Rice University.
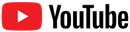
- TPC and eLearning
- Read Watch Interact
- What's NEW at TPC?
- Practice Review Test
- Teacher-Tools
- Subscription Selection
- Seat Calculator
- Ad Free Account
- Edit Profile Settings
- Classes (Version 2)
- Student Progress Edit
- Task Properties
- Export Student Progress
- Task, Activities, and Scores
- Metric Conversions Questions
- Metric System Questions
- Metric Estimation Questions
- Significant Digits Questions
- Proportional Reasoning
- Acceleration
- Distance-Displacement
- Dots and Graphs
- Graph That Motion
- Match That Graph
- Name That Motion
- Motion Diagrams
- Pos'n Time Graphs Numerical
- Pos'n Time Graphs Conceptual
- Up And Down - Questions
- Balanced vs. Unbalanced Forces
- Change of State
- Force and Motion
- Mass and Weight
- Match That Free-Body Diagram
- Net Force (and Acceleration) Ranking Tasks
- Newton's Second Law
- Normal Force Card Sort
- Recognizing Forces
- Air Resistance and Skydiving
- Solve It! with Newton's Second Law
- Which One Doesn't Belong?
- Component Addition Questions
- Head-to-Tail Vector Addition
- Projectile Mathematics
- Trajectory - Angle Launched Projectiles
- Trajectory - Horizontally Launched Projectiles
- Vector Addition
- Vector Direction
- Which One Doesn't Belong? Projectile Motion
- Forces in 2-Dimensions
- Being Impulsive About Momentum
- Explosions - Law Breakers
- Hit and Stick Collisions - Law Breakers
- Case Studies: Impulse and Force
- Impulse-Momentum Change Table
- Keeping Track of Momentum - Hit and Stick
- Keeping Track of Momentum - Hit and Bounce
- What's Up (and Down) with KE and PE?
- Energy Conservation Questions
- Energy Dissipation Questions
- Energy Ranking Tasks
- LOL Charts (a.k.a., Energy Bar Charts)
- Match That Bar Chart
- Words and Charts Questions
- Name That Energy
- Stepping Up with PE and KE Questions
- Case Studies - Circular Motion
- Circular Logic
- Forces and Free-Body Diagrams in Circular Motion
- Gravitational Field Strength
- Universal Gravitation
- Angular Position and Displacement
- Linear and Angular Velocity
- Angular Acceleration
- Rotational Inertia
- Balanced vs. Unbalanced Torques
- Getting a Handle on Torque
- Torque-ing About Rotation
- Properties of Matter
- Fluid Pressure
- Buoyant Force
- Sinking, Floating, and Hanging
- Pascal's Principle
- Flow Velocity
- Bernoulli's Principle
- Balloon Interactions
- Charge and Charging
- Charge Interactions
- Charging by Induction
- Conductors and Insulators
- Coulombs Law
- Electric Field
- Electric Field Intensity
- Polarization
- Case Studies: Electric Power
- Know Your Potential
- Light Bulb Anatomy
- I = ∆V/R Equations as a Guide to Thinking
- Parallel Circuits - ∆V = I•R Calculations
- Resistance Ranking Tasks
- Series Circuits - ∆V = I•R Calculations
- Series vs. Parallel Circuits
- Equivalent Resistance
- Period and Frequency of a Pendulum
- Pendulum Motion: Velocity and Force
- Energy of a Pendulum
- Period and Frequency of a Mass on a Spring
- Horizontal Springs: Velocity and Force
- Vertical Springs: Velocity and Force
- Energy of a Mass on a Spring
- Decibel Scale
- Frequency and Period
- Closed-End Air Columns
- Name That Harmonic: Strings
- Rocking the Boat
- Wave Basics
- Matching Pairs: Wave Characteristics
- Wave Interference
- Waves - Case Studies
- Color Addition and Subtraction
- Color Filters
- If This, Then That: Color Subtraction
- Light Intensity
- Color Pigments
- Converging Lenses
- Curved Mirror Images
- Law of Reflection
- Refraction and Lenses
- Total Internal Reflection
- Who Can See Who?
- Formulas and Atom Counting
- Atomic Models
- Bond Polarity
- Entropy Questions
- Cell Voltage Questions
- Heat of Formation Questions
- Reduction Potential Questions
- Oxidation States Questions
- Measuring the Quantity of Heat
- Hess's Law
- Oxidation-Reduction Questions
- Galvanic Cells Questions
- Thermal Stoichiometry
- Molecular Polarity
- Quantum Mechanics
- Balancing Chemical Equations
- Bronsted-Lowry Model of Acids and Bases
- Classification of Matter
- Collision Model of Reaction Rates
- Density Ranking Tasks
- Dissociation Reactions
- Complete Electron Configurations
- Elemental Measures
- Enthalpy Change Questions
- Equilibrium Concept
- Equilibrium Constant Expression
- Equilibrium Calculations - Questions
- Equilibrium ICE Table
- Intermolecular Forces Questions
- Ionic Bonding
- Lewis Electron Dot Structures
- Limiting Reactants
- Line Spectra Questions
- Mass Stoichiometry
- Measurement and Numbers
- Metals, Nonmetals, and Metalloids
- Metric Estimations
- Metric System
- Molarity Ranking Tasks
- Mole Conversions
- Name That Element
- Names to Formulas
- Names to Formulas 2
- Nuclear Decay
- Particles, Words, and Formulas
- Periodic Trends
- Precipitation Reactions and Net Ionic Equations
- Pressure Concepts
- Pressure-Temperature Gas Law
- Pressure-Volume Gas Law
- Chemical Reaction Types
- Significant Digits and Measurement
- States Of Matter Exercise
- Stoichiometry Law Breakers
- Stoichiometry - Math Relationships
- Subatomic Particles
- Spontaneity and Driving Forces
- Gibbs Free Energy
- Volume-Temperature Gas Law
- Acid-Base Properties
- Energy and Chemical Reactions
- Chemical and Physical Properties
- Valence Shell Electron Pair Repulsion Theory
- Writing Balanced Chemical Equations
- Mission CG1
- Mission CG10
- Mission CG2
- Mission CG3
- Mission CG4
- Mission CG5
- Mission CG6
- Mission CG7
- Mission CG8
- Mission CG9
- Mission EC1
- Mission EC10
- Mission EC11
- Mission EC12
- Mission EC2
- Mission EC3
- Mission EC4
- Mission EC5
- Mission EC6
- Mission EC7
- Mission EC8
- Mission EC9
- Mission RL1
- Mission RL2
- Mission RL3
- Mission RL4
- Mission RL5
- Mission RL6
- Mission KG7
- Mission RL8
- Mission KG9
- Mission RL10
- Mission RL11
- Mission RM1
- Mission RM2
- Mission RM3
- Mission RM4
- Mission RM5
- Mission RM6
- Mission RM8
- Mission RM10
- Mission LC1
- Mission RM11
- Mission LC2
- Mission LC3
- Mission LC4
- Mission LC5
- Mission LC6
- Mission LC8
- Mission SM1
- Mission SM2
- Mission SM3
- Mission SM4
- Mission SM5
- Mission SM6
- Mission SM8
- Mission SM10
- Mission KG10
- Mission SM11
- Mission KG2
- Mission KG3
- Mission KG4
- Mission KG5
- Mission KG6
- Mission KG8
- Mission KG11
- Mission F2D1
- Mission F2D2
- Mission F2D3
- Mission F2D4
- Mission F2D5
- Mission F2D6
- Mission KC1
- Mission KC2
- Mission KC3
- Mission KC4
- Mission KC5
- Mission KC6
- Mission KC7
- Mission KC8
- Mission AAA
- Mission SM9
- Mission LC7
- Mission LC9
- Mission NL1
- Mission NL2
- Mission NL3
- Mission NL4
- Mission NL5
- Mission NL6
- Mission NL7
- Mission NL8
- Mission NL9
- Mission NL10
- Mission NL11
- Mission NL12
- Mission MC1
- Mission MC10
- Mission MC2
- Mission MC3
- Mission MC4
- Mission MC5
- Mission MC6
- Mission MC7
- Mission MC8
- Mission MC9
- Mission RM7
- Mission RM9
- Mission RL7
- Mission RL9
- Mission SM7
- Mission SE1
- Mission SE10
- Mission SE11
- Mission SE12
- Mission SE2
- Mission SE3
- Mission SE4
- Mission SE5
- Mission SE6
- Mission SE7
- Mission SE8
- Mission SE9
- Mission VP1
- Mission VP10
- Mission VP2
- Mission VP3
- Mission VP4
- Mission VP5
- Mission VP6
- Mission VP7
- Mission VP8
- Mission VP9
- Mission WM1
- Mission WM2
- Mission WM3
- Mission WM4
- Mission WM5
- Mission WM6
- Mission WM7
- Mission WM8
- Mission WE1
- Mission WE10
- Mission WE2
- Mission WE3
- Mission WE4
- Mission WE5
- Mission WE6
- Mission WE7
- Mission WE8
- Mission WE9
- Vector Walk Interactive
- Name That Motion Interactive
- Kinematic Graphing 1 Concept Checker
- Kinematic Graphing 2 Concept Checker
- Graph That Motion Interactive
- Two Stage Rocket Interactive
- Rocket Sled Concept Checker
- Force Concept Checker
- Free-Body Diagrams Concept Checker
- Free-Body Diagrams The Sequel Concept Checker
- Skydiving Concept Checker
- Elevator Ride Concept Checker
- Vector Addition Concept Checker
- Vector Walk in Two Dimensions Interactive
- Name That Vector Interactive
- River Boat Simulator Concept Checker
- Projectile Simulator 2 Concept Checker
- Projectile Simulator 3 Concept Checker
- Hit the Target Interactive
- Turd the Target 1 Interactive
- Turd the Target 2 Interactive
- Balance It Interactive
- Go For The Gold Interactive
- Egg Drop Concept Checker
- Fish Catch Concept Checker
- Exploding Carts Concept Checker
- Collision Carts - Inelastic Collisions Concept Checker
- Its All Uphill Concept Checker
- Stopping Distance Concept Checker
- Chart That Motion Interactive
- Roller Coaster Model Concept Checker
- Uniform Circular Motion Concept Checker
- Horizontal Circle Simulation Concept Checker
- Vertical Circle Simulation Concept Checker
- Race Track Concept Checker
- Gravitational Fields Concept Checker
- Orbital Motion Concept Checker
- Angular Acceleration Concept Checker
- Balance Beam Concept Checker
- Torque Balancer Concept Checker
- Aluminum Can Polarization Concept Checker
- Charging Concept Checker
- Name That Charge Simulation
- Coulomb's Law Concept Checker
- Electric Field Lines Concept Checker
- Put the Charge in the Goal Concept Checker
- Circuit Builder Concept Checker (Series Circuits)
- Circuit Builder Concept Checker (Parallel Circuits)
- Circuit Builder Concept Checker (∆V-I-R)
- Circuit Builder Concept Checker (Voltage Drop)
- Equivalent Resistance Interactive
- Pendulum Motion Simulation Concept Checker
- Mass on a Spring Simulation Concept Checker
- Particle Wave Simulation Concept Checker
- Boundary Behavior Simulation Concept Checker
- Slinky Wave Simulator Concept Checker
- Simple Wave Simulator Concept Checker
- Wave Addition Simulation Concept Checker
- Standing Wave Maker Simulation Concept Checker
- Color Addition Concept Checker
- Painting With CMY Concept Checker
- Stage Lighting Concept Checker
- Filtering Away Concept Checker
- InterferencePatterns Concept Checker
- Young's Experiment Interactive
- Plane Mirror Images Interactive
- Who Can See Who Concept Checker
- Optics Bench (Mirrors) Concept Checker
- Name That Image (Mirrors) Interactive
- Refraction Concept Checker
- Total Internal Reflection Concept Checker
- Optics Bench (Lenses) Concept Checker
- Kinematics Preview
- Velocity Time Graphs Preview
- Moving Cart on an Inclined Plane Preview
- Stopping Distance Preview
- Cart, Bricks, and Bands Preview
- Fan Cart Study Preview
- Friction Preview
- Coffee Filter Lab Preview
- Friction, Speed, and Stopping Distance Preview
- Up and Down Preview
- Projectile Range Preview
- Ballistics Preview
- Juggling Preview
- Marshmallow Launcher Preview
- Air Bag Safety Preview
- Colliding Carts Preview
- Collisions Preview
- Engineering Safer Helmets Preview
- Push the Plow Preview
- Its All Uphill Preview
- Energy on an Incline Preview
- Modeling Roller Coasters Preview
- Hot Wheels Stopping Distance Preview
- Ball Bat Collision Preview
- Energy in Fields Preview
- Weightlessness Training Preview
- Roller Coaster Loops Preview
- Universal Gravitation Preview
- Keplers Laws Preview
- Kepler's Third Law Preview
- Charge Interactions Preview
- Sticky Tape Experiments Preview
- Wire Gauge Preview
- Voltage, Current, and Resistance Preview
- Light Bulb Resistance Preview
- Series and Parallel Circuits Preview
- Thermal Equilibrium Preview
- Linear Expansion Preview
- Heating Curves Preview
- Electricity and Magnetism - Part 1 Preview
- Electricity and Magnetism - Part 2 Preview
- Vibrating Mass on a Spring Preview
- Period of a Pendulum Preview
- Wave Speed Preview
- Slinky-Experiments Preview
- Standing Waves in a Rope Preview
- Sound as a Pressure Wave Preview
- DeciBel Scale Preview
- DeciBels, Phons, and Sones Preview
- Sound of Music Preview
- Shedding Light on Light Bulbs Preview
- Models of Light Preview
- Electromagnetic Radiation Preview
- Electromagnetic Spectrum Preview
- EM Wave Communication Preview
- Digitized Data Preview
- Light Intensity Preview
- Concave Mirrors Preview
- Object Image Relations Preview
- Snells Law Preview
- Reflection vs. Transmission Preview
- Magnification Lab Preview
- Reactivity Preview
- Ions and the Periodic Table Preview
- Periodic Trends Preview
- Intermolecular Forces Preview
- Melting Points and Boiling Points Preview
- Reaction Rates Preview
- Ammonia Factory Preview
- Stoichiometry Preview
- Nuclear Chemistry Preview
- Gaining Teacher Access
- Tasks and Classes
- Tasks - Classic
- Subscription
- Subscription Locator
- 1-D Kinematics
- Newton's Laws
- Vectors - Motion and Forces in Two Dimensions
- Momentum and Its Conservation
- Work and Energy
- Circular Motion and Satellite Motion
- Thermal Physics
- Static Electricity
- Electric Circuits
- Vibrations and Waves
- Sound Waves and Music
- Light and Color
- Reflection and Mirrors
- About the Physics Interactives
- Task Tracker
- Usage Policy
- Newtons Laws
- Vectors and Projectiles
- Forces in 2D
- Momentum and Collisions
- Circular and Satellite Motion
- Balance and Rotation
- Electromagnetism
- Waves and Sound
- Atomic Physics
- Forces in Two Dimensions
- Work, Energy, and Power
- Circular Motion and Gravitation
- Sound Waves
- 1-Dimensional Kinematics
- Circular, Satellite, and Rotational Motion
- Einstein's Theory of Special Relativity
- Waves, Sound and Light
- QuickTime Movies
- About the Concept Builders
- Pricing For Schools
- Directions for Version 2
- Measurement and Units
- Relationships and Graphs
- Rotation and Balance
- Vibrational Motion
- Reflection and Refraction
- Teacher Accounts
- Task Tracker Directions
- Kinematic Concepts
- Kinematic Graphing
- Wave Motion
- Sound and Music
- About CalcPad
- 1D Kinematics
- Vectors and Forces in 2D
- Simple Harmonic Motion
- Rotational Kinematics
- Rotation and Torque
- Rotational Dynamics
- Electric Fields, Potential, and Capacitance
- Transient RC Circuits
- Light Waves
- Units and Measurement
- Stoichiometry
- Molarity and Solutions
- Thermal Chemistry
- Acids and Bases
- Kinetics and Equilibrium
- Solution Equilibria
- Oxidation-Reduction
- Nuclear Chemistry
- NGSS Alignments
- 1D-Kinematics
- Projectiles
- Circular Motion
- Magnetism and Electromagnetism
- Graphing Practice
- About the ACT
- ACT Preparation
- For Teachers
- Other Resources
- Newton's Laws of Motion
- Work and Energy Packet
- Static Electricity Review
- Solutions Guide
- Solutions Guide Digital Download
- Motion in One Dimension
- Work, Energy and Power
- Frequently Asked Questions
- Purchasing the Download
- Purchasing the CD
- Purchasing the Digital Download
- About the NGSS Corner
- NGSS Search
- Force and Motion DCIs - High School
- Energy DCIs - High School
- Wave Applications DCIs - High School
- Force and Motion PEs - High School
- Energy PEs - High School
- Wave Applications PEs - High School
- Crosscutting Concepts
- The Practices
- Physics Topics
- NGSS Corner: Activity List
- NGSS Corner: Infographics
- About the Toolkits
- Position-Velocity-Acceleration
- Position-Time Graphs
- Velocity-Time Graphs
- Newton's First Law
- Newton's Second Law
- Newton's Third Law
- Terminal Velocity
- Projectile Motion
- Forces in 2 Dimensions
- Impulse and Momentum Change
- Momentum Conservation
- Work-Energy Fundamentals
- Work-Energy Relationship
- Roller Coaster Physics
- Satellite Motion
- Electric Fields
- Circuit Concepts
- Series Circuits
- Parallel Circuits
- Describing-Waves
- Wave Behavior Toolkit
- Standing Wave Patterns
- Resonating Air Columns
- Wave Model of Light
- Plane Mirrors
- Curved Mirrors
- Teacher Guide
- Using Lab Notebooks
- Current Electricity
- Light Waves and Color
- Reflection and Ray Model of Light
- Refraction and Ray Model of Light
- Classes (Legacy Version)
- Teacher Resources
- Subscriptions

- Newton's Laws
- Einstein's Theory of Special Relativity
- About Concept Checkers
- School Pricing
- Newton's Laws of Motion
- Newton's First Law
- Newton's Third Law
- Traveling Waves vs. Standing Waves
- Formation of Standing Waves
- Nodes and Anti-nodes
- Harmonics and Patterns
- Mathematics of Standing Waves
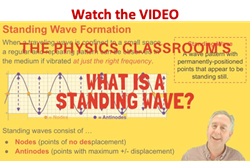
What is a Standing Wave Pattern?
It is however possible to have a wave confined to a given space in a medium and still produce a regular wave pattern that is readily discernible amidst the motion of the medium. For instance, if an elastic rope is held end-to-end and vibrated at just the right frequency , a wave pattern would be produced that assumes the shape of a sine wave and is seen to change over time. The wave pattern is only produced when one end of the rope is vibrated at just the right frequency. When the proper frequency is used, the interference of the incident wave and the reflected wave occur in such a manner that there are specific points along the medium that appear to be standing still. Because the observed wave pattern is characterized by points that appear to be standing still, the pattern is often called a standing wave pattern . There are other points along the medium whose displacement changes over time, but in a regular manner. These points vibrate back and forth from a positive displacement to a negative displacement; the vibrations occur at regular time intervals such that the motion of the medium is regular and repeating. A pattern is readily observable.
We Would Like to Suggest ...
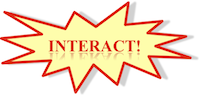
Revision notes for IB Physics
Topic 4: waves.
See the guide for this topic.
4.1 – Oscillations
Simple harmonic oscillations.
Oscillations are periodic motions which center around an equilibrium position.
Simple harmonic motion (SHM) is a special type of oscillation. For example:
- The simple pendulum
- The vibration of strings in a violin
- The spring-mass system, where the mass is initially displaced to produce a periodic motion around the equilibrium position
An object undergoes SHM if it experiences a force which is proportional and opposite of the displacement from its equilibrium position.
for a pendulum
for a spring-mass system
Time period, frequency, amplitude, displacement and phase difference

Conditions for simple harmonic motion
- When the body is displaced from equilibrium, there must exist a restoring force (a force that wants to pull the body back to equilibrium).
- The magnitude of the restoring force must be proportional to the displacement of the body and acts towards the equilibrium.
4.2 – Travelling waves
Travelling waves.
A travelling wave is a continuous disturbance in a medium characterized by repeating oscillations. For example:
- A rope that is flicked up and down continuously creates a repeating disturbance similar to the shape of a sine/cosine wave.
Energy is transferred by waves.
Matter is not transferred by waves.
The direction of a wave is defined by the direction of the energy transfer.
Wavelength, frequency, period and wave speed
Wavelength, frequency, and period follow the same rules of SHM.
Wave speed can be calculated by the following equation

Transverse and longitudinal waves
The nature of electromagnetic waves.
All EM waves travel in vacuum at the same speed of 3*10^8m/s.
EM waves are transverse waves.

The nature of sound waves
The speed of sound in 20 degrees Celsius dry air is approximately 343.2m/s.
Sound waves are longitudinal waves.
4.3 – Wave characteristics
Wavefronts and rays.
Wavefronts:
- Lines joining points which vibrate in phase.
- Can be straight lines or curves.
- The distance between successive wavefronts is the wavelength of the wave.
- Lines which indicate the direction of wave propagation.
- Rays are perpendicular to wavefronts.

Amplitude and intensity
The amplitude and intensity of a wave depends on its energy.
The intensity of a wave is proportional to the square of its amplitude (I∝A^2).

See previous section with the same title.
Superposition
The principle of superposition states that the net displacement of the underlying medium for a wave is equal to the sum of the individual wave displacements.

The left shows constructive interference (superposition) where the two waves add up (e.g. 1+1=2). The right shows deconstructive interference (superposition) where the two waves cancel each other (e.g. 1+(-1)=0).
Polarization
Light is a transverse wave (polarization only occur to transverse waves).
The polarization of light refers to the orientation of the oscillation in the underlying electric field.
Light is plane polarized if the electric field oscillates in one plane.

Left shows unpolarized light and right shows polarized light.
Polarization by reflection
When light is transmitted across a boundary between two mediums with different refractive indexes, part of the light is reflected and the remaining part is refracted (for further explanation, see section 4.4).
The light reflected is partially polarized, meaning that it is a mixture of polarized light and unpolarized light.
The extent to which the reflected light is polarized depends on the angle of incidence and the refractive index of the two mediums.
The angle of incidence at which the reflected light is totally polarized is called the Brewster’s angle (ϕ) given by the equation
where n1 and n2 are the refractive indexes for their respective mediums
When the angle of incidence is equal to Brewster’s angle, the reflected ray is totally polarized and the reflected ray is perpendicular to the refracted ray.

Polarizers and Analyzers
- A polarizer is a sheet of material which polarizes light.
- When unpolarized light passes through a polarizer, its intensity is reduced by 50%.
- When polarized light passes through a polarizer, its intensity will be reduced by a factor dependent on the orientation of the polarizer. This property allows us to deduce the polarization of light by using a polarizer.
- A polarizer used for this purpose is called an analyzer.
Malus’ Law relates the incident intensity and transmitted intensity of light passing through a polarizer and an analyzer.

where I is the transmitted intensity, I0 is the initial light intensity upon the analyzer, θ is the angle between the transmission axis and the analyzer.
When light passes through an optically active substance, the plane of polarization rotates.
4.4 – Wave behaviour
Reflection and refraction.
Angle of incidence = Angle of reflection
Reflection of waves from a fixed end is inverted.
Reflection of waves from a free end is not inverted.
Refraction is the change in direction of a wave when it transmits from one medium to another.

The angle of incidence and the angle of refraction can be determined by Snell’s law given by the following formula

Fast-to-slow: towards normal; slow-to-fast: away from normal
In addition, the refractive index n1 and n2 are related by the following equation

where v1 and v2 are the speed of the waves in their respective mediums and λ1 and λ2 are the wavelength of the waves of their respective mediums
Snell’s law, critical angle and total internal reflection
See previous section (Reflection and refraction) for Snell’s law.

Diffraction through a single-slit and around objects
- Diffraction through a single-slit
Single-slit equations are not required for the standard level course.
- Diffraction around objects

Interference patterns
Maximums form at constructive interference (the maximum is shown by 1-2) and minimums form at deconstructive interference (the first minimum is shown by 3-4).

Double-slit interference
Like single-slit diffraction, double-slit diffraction occurs via the same methods of interference and has a similar diffraction pattern.

Path difference

4.5 – Standing waves
The nature of standing waves.
Standing waves (stationary) waves result from the superposition of two opposite waves which are otherwise identical.
Energy is not transferred by standing waves.
A wave hits a wall and is reflected identically opposite.
The black wave shows the wave created by the superposition of the blue and green waves.
Boundary conditions
Air particles can oscillate and create standing waves in pipes with open or closed ends.
Antinodes are positioned at open ends and nodes are positioned at closed ends.
Standing waves on a string is equivalent to that in a pipe which is closed on both ends (nodes-node).

The following table summarizes the behavior of standing waves in pipes and strings:
Nodes and antinodes
Positions along the wave which are fixed are called nodes (minimum) and those with the largest displacement are called antinodes (maximum).
For standing waves, the distance between adjacent nodes = the distance between adjacent antinodes = λ/2.
Difference between standing waves and travelling waves
Share this:
Travelling waves
Shifting the position, turning standing waves into travelling waves, another way to make waves travel, the speed of a wave on a string, for more information.
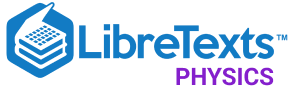
- school Campus Bookshelves
- menu_book Bookshelves
- perm_media Learning Objects
- login Login
- how_to_reg Request Instructor Account
- hub Instructor Commons
- Download Page (PDF)
- Download Full Book (PDF)
- Periodic Table
- Physics Constants
- Scientific Calculator
- Reference & Cite
- Tools expand_more
- Readability
selected template will load here
This action is not available.
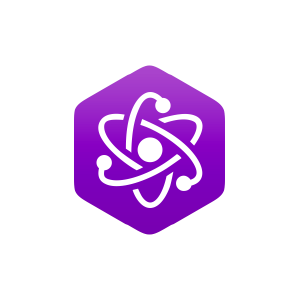
14.1: Characteristics of a wave
- Last updated
- Save as PDF
- Page ID 19461
Definition and types of waves
A traveling wave is a disturbance that travels through a medium. Consider the waves made by fans at a soccer game, as in Figure \(\PageIndex{1}\). The fans can be thought of as the medium through which the wave propagates. The elements of the medium may oscillate about an equilibrium position (the fans move a short distance up and down), but they do not travel with the wave (the fans do not move horizontally with the wave).
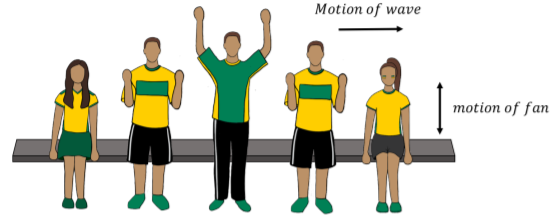
Consider the ripples (waves) made by a rock dropped in a pond (Figure \(\PageIndex{2}\)). The ripples travel outwards from where the rock was dropped, but the water itself does not move outwards. The individual water molecules will move in small circles about an equilibrium position, but they do not move along with the waves.
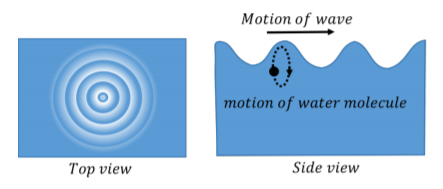
We can distinguish between two classes of waves, based on the motion of the medium through which it propagates. With transverse waves , the elements of the medium oscillate back and forth in a direction perpendicular to the motion of the wave. For example, if you attach a horizontal rope to a wall and move the other end up and down (Figure \(\PageIndex{3}\)), you can create a disturbance (a wave) that travels horizontally along the rope. The parts of the rope do not move horizontally; they only move up and down, about some equilibrium position.
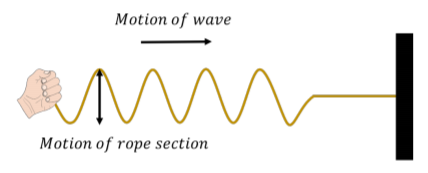
With longitudinal waves , the elements of the medium oscillate back and forth in the same direction as the motion of the wave. If you clap your hands, you will create a pressure disturbance in the air that will propagate; this is what we call sound (a sound wave). The air molecules oscillate about an equilibrium position in the same direction as the wave propagates, but they do not move with the wave.
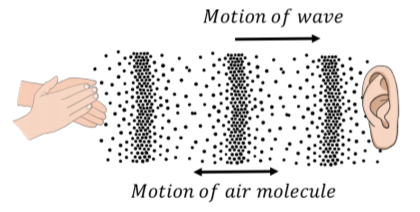
Furthermore, we can distinguish between “travelling waves”, in which a disturbance propagates through a medium, and “standing waves”, which do not transport energy through the medium (for example, a vibrating string on a violin).
Exercise \(\PageIndex{1}\)
Are the waves propagating through a slinky when you compress and elongate it (Figure \(\PageIndex{5}\)) transverse or longitudinal?

- Longitudinal
Physically, a wave can only propagate through a medium if the medium can be deformed. When a particle in the medium is disturbed from its equilibrium position, it will experience a restoring force that acts to bring it back to its equilibrium position. Often, if the displacement of the particle from the equilibrium is small, the magnitude of that force is proportional to the displacement. Thus, as we will see, we can model the propagation of waves by treating the particles in the medium as simple harmonic oscillators.
A source of energy is required in order to deform the medium and generate a wave. For example, that source of energy could be a speaker creating sound waves by pushing a membrane back and forth; speakers require energy, and are often rated by the electrical power that they convert into sound waves (e.g. a \(50\text{W}\) speaker consumes \(50\text{W}\) of electrical power to produce sound).
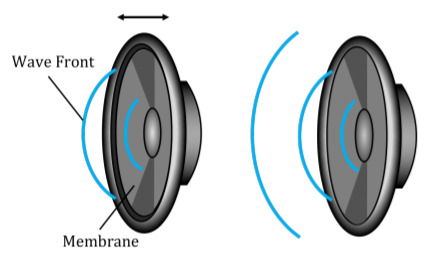
Description of a wave
In this chapter, we will mostly discuss how to describe sinusoidal waves; those for which the displacement of particles in the medium can be described by a sinusoidally-varying function of position. As we will see, more complicated waves can always be described as if they are the combination of multiple sine waves. We can use several quantities to describe a traveling wave, which are illustrated in Figure \(\PageIndex{7}\):
- The wavelength , \(\lambda\) , is the distance between two successive maxima (“peaks”) or minima (“troughs”) in the wave.
- The amplitude , \(A\) , is the maximal distance that a particle in the medium is displaced from its equilibrium position.
- The velocity , \(\vec v\) , is the velocity with which the disturbance propagates through the medium.
- The period , \(T\) , is the time it takes for two successive maxima (or minima) to pass through the same point in the medium.
- The frequency , \(f\) , is the inverse of the period ( \(f=1/T\) ).
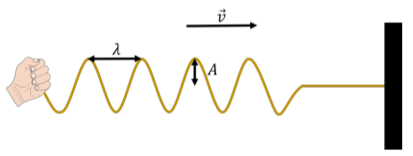
The wavelength, speed, and period of the wave are related, since the amount of time that it takes for two successive maxima of the wave to pass through a given point will depend on the speed of the wave and the distance between maxima, \(\lambda\) . Since it takes a time, \(T\) , for two maxima a distance \(\lambda\) apart to pass through a given point in the medium, the speed of the wave is given by:
\[v=\frac{\lambda}{T}=\lambda f\]
Thus, of the three quantities (speed, period/frequency, and wavelength), only two are independent, as the third quantity must depend on the value of the other two. The speed of a wave depends on the properties of the medium through which the wave propagates and not on the mechanism that is generating the wave . For example, the speed of sound waves depends on the pressure, density, and temperature of the air through which they propagate, and not on what is making the sound. When a mechanism generates a wave, that mechanism usually determines the frequency of the wave (e.g. frequency with which the hand in Figure \(\PageIndex{7}\) moves up and down), the speed is determined by the medium, and the wavelength can be determined from Equation 14.1.1 .
Exercise \(\PageIndex{2}\)
What can you say about the sound emitted by a cello versus that emitted by a violin?
- The sound from the violin has a higher frequency.
- The sound from the cello has a longer wavelength.
- The sound from both instruments propagates at the same speed.
- All of the above.
Thank you for visiting nature.com. You are using a browser version with limited support for CSS. To obtain the best experience, we recommend you use a more up to date browser (or turn off compatibility mode in Internet Explorer). In the meantime, to ensure continued support, we are displaying the site without styles and JavaScript.
- View all journals
- Explore content
- About the journal
- Publish with us
- Sign up for alerts
- Review Article
- Published: 22 March 2018
Cortical travelling waves: mechanisms and computational principles
- Lyle Muller 1 ,
- Frédéric Chavane 2 ,
- John Reynolds 1 &
- Terrence J. Sejnowski 1 , 3
Nature Reviews Neuroscience volume 19 , pages 255–268 ( 2018 ) Cite this article
20k Accesses
257 Citations
175 Altmetric
Metrics details
- Dynamical systems
- Neural encoding
- Visual system
Multichannel recording technologies have revealed travelling waves of neural activity in multiple sensory, motor and cognitive systems. These waves can be spontaneously generated by recurrent circuits or evoked by external stimuli. They travel along brain networks at multiple scales, transiently modulating spiking and excitability as they pass. Here, we review recent experimental findings that have found evidence for travelling waves at single-area (mesoscopic) and whole-brain (macroscopic) scales. We place these findings in the context of the current theoretical understanding of wave generation and propagation in recurrent networks. During the large low-frequency rhythms of sleep or the relatively desynchronized state of the awake cortex, travelling waves may serve a variety of functions, from long-term memory consolidation to processing of dynamic visual stimuli. We explore new avenues for experimental and computational understanding of the role of spatiotemporal activity patterns in the cortex.
This is a preview of subscription content, access via your institution
Access options
Access Nature and 54 other Nature Portfolio journals
Get Nature+, our best-value online-access subscription
24,99 € / 30 days
cancel any time
Subscribe to this journal
Receive 12 print issues and online access
176,64 € per year
only 14,72 € per issue
Buy this article
- Purchase on Springer Link
- Instant access to full article PDF
Prices may be subject to local taxes which are calculated during checkout
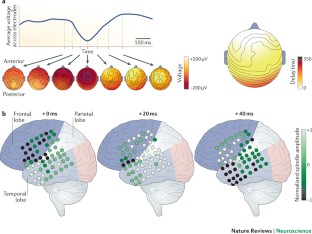
Similar content being viewed by others
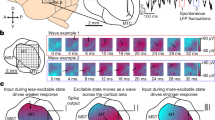
Spontaneous travelling cortical waves gate perception in behaving primates
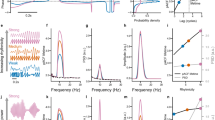
Rhythmicity of neuronal oscillations delineates their cortical and spectral architecture
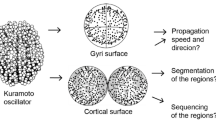
Cortical traveling waves reflect state-dependent hierarchical sequencing of local regions in the human connectome network
von der Malsburg, C. The Correlation Theory of Brain Function (Max-Planck-Institute for Biophysical Chemistry, Gottingen, Germany, 1981).
Google Scholar
Gray, C. M. & Singer, W. Stimulus-specific neuronal oscillations in orientation columns of cat visual cortex. Proc. Natl Acad. Sci. USA 86 , 1698–1702 (1989).
Article CAS PubMed PubMed Central Google Scholar
Salinas, E. & Sejnowski, T. J. Correlated neuronal activity and the flow of neural information. Nat. Rev. Neurosci. 2 , 539–550 (2001).
Akam, T. & Kullmann, D. M. Oscillations and filtering networks support flexible routing of information. Neuron 67 , 308–320 (2010).
Swadlow, H. A., Beloozerova, I. N. & Sirota, M. G. Sharp, local synchrony among putative feed-forward inhibitory interneurons of rabbit somatosensory cortex. J. Neurophysiol. 79 , 567–582 (1998).
Article CAS PubMed Google Scholar
Usrey, W. M. & Reid, R. C. Synchronous activity in the visual system. Annu. Rev. Physiol. 61 , 435–456 (1999).
Aertsen, A. M., Gerstein, G. L., Habib, M. K. & Palm, G. Dynamics of neuronal firing correlation: modulation of' effective connectivity'. J. Neurophysiol. 61 , 900–917 (1989).
Sohal, V. S., Zhang, F., Yizhar, O. & Deisseroth, K. Parvalbumin neurons and gamma rhythms enhance cortical circuit performance. Nature 459 , 698–702 (2009).
Huerta, P. T. & Lisman, J. E. Bidirectional synaptic plasticity induced by a single burst during cholinergic theta oscillation in CA1 in vitro. Neuron 15 , 1053–1063 (1995).
Ni, J. et al. Gamma-rhythmic gain modulation. Neuron 92 , 240–251 (2016).
Gray, C. M., König, P., Engel, A. K. & Singer, W. Oscillatory responses in cat visual cortex exhibit inter-columnar synchronization which reflects global stimulus properties. Nature 338 , 334–337 (1989).
Uhlhaas, P. J. et al. Neural synchrony in cortical networks: history, concept and current status. Front. Integr. Neurosci. 3 , 17 (2009).
Article PubMed PubMed Central Google Scholar
Muller, L. et al. Rotating waves during human sleep spindles organize global patterns of activity that repeat precisely through the night. eLife 5 , e17267 (2016). This analysis of ECoG recordings from human clinical patients reveals that the 11–15 Hz sleep spindle, long studied for its connection to learning and memory, is robustly organized into rotating waves travelling in a specific direction (from temporal to parietal to frontal cortex).
Muller, L., Reynaud, A., Chavane, F. & Destexhe, A. The stimulus-evoked population response in visual cortex of awake monkey is a propagating wave. Nat. Commun. 5 , 3675 (2014). This paper showed that, during the awake state, small visual stimuli systematically evoke travelling waves extending far beyond the feedforward input in V1 and V2 of macaques.
Article PubMed Google Scholar
Skaggs, W. E., McNaughton, B. L., Wilson, M. A. & Barnes, C. A. Theta phase precession in hippocampal neuronal populations and the compression of temporal sequences. Hippocampus 6 , 149–172 (1996).
3.0.CO;2-K" data-track-action="article reference" href="https://doi.org/10.1002%2F%28SICI%291098-1063%281996%296%3A2%3C149%3A%3AAID-HIPO6%3E3.0.CO%3B2-K" aria-label="Article reference 15" data-doi="10.1002/(SICI)1098-1063(1996)6:2 3.0.CO;2-K">Article CAS PubMed Google Scholar
Tsodyks, M., Kenet, T., Grinvald, A. & Arieli, A. Linking spontaneous activity of single cortical neurons and the underlying functional architecture. Science 286 , 1943–1946 (1999).
Lubenov, E. V. & Siapas, A. G. Hippocampal theta oscillations are travelling waves. Nature 459 , 534–539 (2009). This study showed that theta oscillations, long thought to be approximately synchronous throughout the hippocampus, are in fact travelling waves.
Patel, J., Fujisawa, S., Berényi, A., Royer, S. & Buzsáki, G. Traveling theta waves along the entire septotemporal axis of the hippocampus. Neuron 75 , 410–417 (2012).
Takahashi, K. et al. Large-scale spatiotemporal spike patterning consistent with wave propagation in motor cortex. Nat. Commun. 6 , 7169 (2015). This paper specifically demonstrates that travelling waves modulate spiking activity in the motor cortex during normal waking behaviour.
Arieli, A., Sterkin, A., Grinvald, A. & Aertsen, A. Dynamics of ongoing activity: explanation of the large variability in evoked cortical responses. Science 273 , 1868–1871 (1996).
Nauhaus, I., Busse, L., Carandini, M. & Ringach, D. L. Stimulus contrast modulates functional connectivity in visual cortex. Nat. Neurosci. 12 , 70–76 (2009). This study in anaesthetized cats and monkeys suggests that evoked travelling waves detected with spike-triggered LFP become less visible in high-input regimes; however, recent work has shown that travelling waves persist during high-input regimes and the awake state.
Sato, T. K., Nauhaus, I. & Carandini, M. Traveling waves in visual cortex. Neuron 75 , 218–229 (2012).
Grinvald, A., Lieke, E. E., Frostig, R. D. & Hildesheim, R. Cortical point-spread function and long-range lateral interactions revealed by real-time optical imaging of macaque monkey primary visual cortex. J. Neurosci. 14 , 2545–2568 (1994). This demonstrated the application of VSD in anaesthetized monkeys; using local stimuli, this study demonstrates that the cortical point spread function extends beyond the imprint of the feedforward input, with gradually increasing latency.
Jancke, D., Chavane, F., Naaman, S. & Grinvald, A. Imaging cortical correlates of illusion in early visual cortex. Nature 428 , 423–426 (2004). This study showed that intracortical propagation can give rise to a coherently moving pattern of cortical activity from a sequence of two static stimuli.
Chavane, F. et al. Lateral spread of orientation selectivity in V1 is controlled by intracortical cooperativity. Front. Syst. Neurosci. 5 , 4 (2011).
PubMed PubMed Central Google Scholar
Roland, P. E. et al. Cortical feedback depolarization waves: a mechanism of top-down influence on early visual areas. Proc. Natl Acad. Sci. USA 103 , 12586–12591 (2006).
Petersen, C. C. H., Hahn, T. T. G., Mehta, M., Grinvald, A. & Sakmann, B. Interaction of sensory responses with spontaneous depolarization in layer 2/3 barrel cortex. Proc. Natl Acad. Sci. USA 100 , 13638–13643 (2003).
Ferezou, I., Bolea, S. & Petersen, C. C. H. Visualizing the cortical representation of whisker touch: voltage-sensitive dye imaging in freely moving mice. Neuron 50 , 617–629 (2006).
Zanos, T. P., Mineault, P. J., Nasiotis, K. T., Guitton, D. & Pack, C. C. A sensorimotor role for traveling waves in primate visual cortex. Neuron 85 , 615–627 (2015). This study tied travelling waves in the sensory cortex to a sensorimotor behaviour — visual saccades.
Besserve, M., Lowe, S. C., Logothetis, N. K., Schölkopf, B. & Panzeri, S. Shifts of gamma phase across primary visual cortical sites reflect dynamic stimulus-modulated information transfer. PLoS Biol. 13 , e1002257 (2015). Using an advanced information-theoretic analysis in recordings from anaesthetized monkeys, this study demonstrates that movie stimuli evoke complex spatiotemporal patterns consistent with propagation of activity along the horizontal-fibre network in V1.
Wu, J.-Y., Huang, X. & Zhang, C. Propagating waves of activity in the neocortex: what they are, what they do. Neuroscientist 14 , 487–502 (2008).
Muller, L. & Destexhe, A. Propagating waves in thalamus, cortex and the thalamocortical system: Experiments and models. J. Physiol. 106 , 222–238 (2012).
Hebb, D. O. The Organization of Behavior: A Neuropsychological Theory . (Psychology Press, 2005).
Book Google Scholar
Sejnowski, T. J. The book of Hebb. Neuron 24 , 773–776 (1999).
Swadlow, H. A. & Waxman, S. G. Axonal conduction delays. Scholarpedia 7 , 1451 (2012).
Article Google Scholar
Maynard, E. M., Nordhausen, C. T. & Normann, R. A. The Utah intracortical electrode array: a recording structure for potential brain-computer interfaces. Electroencephalogr. Clin. Neurophysiol. 102 , 228–239 (1997).
Shoham, D. et al. Imaging cortical dynamics at high spatial and temporal resolution with novel blue voltage-sensitive dyes. Neuron 24 , 791–802 (1999).
Girard, P., Hupé, J. M. & Bullier, J. Feedforward and feedback connections between areas V1 and V2 of the monkey have similar rapid conduction velocities. J. Neurophysiol. 85 , 1328–1331 (2001).
Ahmed, O. J. & Cash, S. S. Finding synchrony in the desynchronized EEG: the history and interpretation of gamma rhythms. Front. Integr. Neurosci. 7 , 58 (2013).
Berger, H. Uber das Elektrenkephalogramm des Menschen (1929).
Hughes, J. R. The phenomenon of travelling waves: a review. Clin. Electroencephalogr. 26 , 1–6 (1995).
Hindriks, R., van Putten, M. J. A. M. & Deco, G. Intra-cortical propagation of EEG alpha oscillations. Neuroimage 103 , 444–453 (2014).
van Ede, F., van Pelt, S., Fries, P. & Maris, E. Both ongoing alpha and visually induced gamma oscillations show reliable diversity in their across-site phase-relations. J. Neurophysiol. 113 , 1556–1563 (2015).
Massimini, M., Huber, R., Ferrarelli, F., Hill, S. & Tononi, G. The sleep slow oscillation as a traveling wave. J. Neurosci. 24 , 6862–6870 (2004).
Ribary, U. et al. Magnetic field tomography of coherent thalamocortical 40-Hz oscillations in humans. Proc. Natl Acad. Sci. USA 88 , 11037–11041 (1991). With a novel technique for analysing data from magnetoencephalography, this study reports a unique, global anterior-to-posterior phase shift in gamma oscillations in the human cortex.
Buzsáki, G., Anastassiou, C. A. & Koch, C. The origin of extracellular fields and currents — EEG, ECoG, LFP and spikes. Nat. Rev. Neurosci. 13 , 407–420 (2012).
Nunez, P. L. & Srinivasan, R. Electric Fields of the Brain: The Neurophysics of EEG . (Oxford Univ. Press, 2006).
Bédard, C. & Destexhe, A. Mean-field formulation of Maxwell equations to model electrically inhomogeneous and isotropic media. JEMAA 06 , 296–302 (2014).
Bedard, C., Gomes, J.-M., Bal, T. & Destexhe, A. A framework to reconcile frequency scaling measurements, from intracellular recordings, local-field potentials, up to EEG and MEG signals. J. Integr. Neurosci. 16 , 3–18 (2017).
Alexander, D. M. et al. Traveling waves and trial averaging: the nature of single-trial and averaged brain responses in large-scale cortical signals. Neuroimage 73 , 95–112 (2013). This study details how trial averaging can shape evoked responses and observed spatiotemporal dynamics in macroscopic recordings of the human brain.
Jasper, H. & Penfield, W. Electrocorticograms in man: Effect of voluntary movement upon the electrical activity of the precentral gyrus. Arch. Psychiatr. Nervenkr. Z. Gesamte Neurol. Psychiatr. 183 , 163–174 (1949).
Khodagholy, D. et al. NeuroGrid: recording action potentials from the surface of the brain. Nat. Neurosci. 18 , 310–315 (2015).
Muller, L., Hamilton, L. S., Edwards, E., Bouchard, K. E. & Chang, E. F. Spatial resolution dependence on spectral frequency in human speech cortex electrocorticography. J. Neural Eng. 13 , 056013 (2016).
Ray, S. & Maunsell, J. H. R. Different origins of gamma rhythm and high-gamma activity in macaque visual cortex. PLoS Biol. 9 , e1000610 (2011).
Bahramisharif, A. et al. Propagating neocortical gamma bursts are coordinated by traveling alpha waves. J. Neurosci. 33 , 18849–18854 (2013).
Hangya, B. et al. Complex propagation patterns characterize human cortical activity during slow-wave sleep. J. Neurosci. 31 , 8770–8779 (2011).
Mak-McCully, R. A. et al. Distribution, amplitude, incidence, co-occurrence, and propagation of human K-complexes in focal transcortical recordings. eNeuro https://doi.org/10.1523/ENEURO.0028-15.2015 (2015).
Nir, Y. et al. Regional slow waves and spindles in human sleep. Neuron 70 , 153–169 (2011).
Andrillon, T. et al. Sleep spindles in humans: insights from intracranial EEG and unit recordings. J. Neurosci. 31 , 17821–17834 (2011).
Schüz, A. & Braitenberg, V. in Cortical Areas: Unity and Diversity (ed Schüz, A. & Miller, R.) 377–385 (Taylor Francis, London, 2002).
Reid, R. C. & Alonso, J. M. Specificity of monosynaptic connections from thalamus to visual cortex. Nature 378 , 281–284 (1995).
Ferster, D., Chung, S. & Wheat, H. Orientation selectivity of thalamic input to simple cells of cat visual cortex. Nature 380 , 249–252 (1996).
Chung, S. & Ferster, D. Strength and orientation tuning of the thalamic input to simple cells revealed by electrically evoked cortical suppression. Neuron 20 , 1177–1189 (1998).
Douglas, R. J., Koch, C., Mahowald, M., Martin, K. A. & Suarez, H. H. Recurrent excitation in neocortical circuits. Science 269 , 981–985 (1995).
Sompolinsky, H. & Shapley, R. New perspectives on the mechanisms for orientation selectivity. Curr. Opin. Neurobiol. 7 , 514–522 (1997).
Prechtl, J. C., Cohen, L. B., Pesaran, B., Mitra, P. P. & Kleinfeld, D. Visual stimuli induce waves of electrical activity in turtle cortex. Proc. Natl Acad. Sci. USA 94 , 7621–7626 (1997).
Bringuier, V., Chavane, F., Glaeser, L. & Frégnac, Y. Horizontal propagation of visual activity in the synaptic integration field of area 17 neurons. Science 283 , 695–699 (1999). This study provided experimental evidence for evoked travelling waves in the mammalian cortex and uses intracellular recordings in which subthreshold depolarizing responses outside the spiking receptive field become more delayed for stimuli presented farther from the receptive field centre.
Slovin, H., Arieli, A., Hildesheim, R. & Grinvald, A. Long-term voltage-sensitive dye imaging reveals cortical dynamics in behaving monkeys. J. Neurophysiol. 88 , 3421–3438 (2002).
Xu, W., Huang, X., Takagaki, K. & Wu, J.-Y. Compression and reflection of visually evoked cortical waves. Neuron 55 , 119–129 (2007).
Han, F., Caporale, N. & Dan, Y. Reverberation of recent visual experience in spontaneous cortical waves. Neuron 60 , 321–327 (2008). This is the first study to show dynamic changes in the pattern of spontaneous travelling waves in the cortex following repeated sensory stimulation, which is shown in anaesthetized rodents.
Ray, S. & Maunsell, J. H. R. Network rhythms influence the relationship between spike-triggered local field potential and functional connectivity. J. Neurosci. 31 , 12674–12682 (2011).
Nauhaus, I., Busse, L., Ringach, D. L. & Carandini, M. Robustness of traveling waves in ongoing activity of visual cortex. J. Neurosci. 32 , 3088–3094 (2012).
Telenczuk, B. & Destexhe, A. How neuronal correlations affect the LFP signal? BMC Neurosci. 16 , 60 (2015).
Sit, Y. F., Chen, Y., Geisler, W. S., Miikkulainen, R. & Seidemann, E. Complex dynamics of V1 population responses explained by a simple gain-control model. Neuron 64 , 943–956 (2009).
Maris, E., Womelsdorf, T., Desimone, R. & Fries, P. Rhythmic neuronal synchronization in visual cortex entails spatial phase relation diversity that is modulated by stimulation and attention. Neuroimage 74 , 99–116 (2013).
Maris, E., Fries, P. & van Ede, F. Diverse phase relations among neuronal rhythms and their potential function. Trends Neurosci. 39 , 86–99 (2016).
Gabriel, A. & Eckhorn, R. A multi-channel correlation method detects traveling γ-waves in monkey visual cortex. J. Neurosci. Methods 131 , 171–184 (2003). This study reported that gamma oscillations in V1 can be organized into travelling waves in awake macaques.
Freeman, W. J. & Barrie, J. M. Analysis of spatial patterns of phase in neocortical gamma EEGs in rabbit. J. Neurophysiol. 84 , 1266–1278 (2000).
Vinck, M. et al. Gamma-phase shifting in awake monkey visual cortex. J. Neurosci. 30 , 1250–1257 (2010).
Hubel, D. H. & Wiesel, T. N. Receptive fields of single neurones in the cat's striate cortex. J. Physiol. 148 , 574–591 (1959).
Hubel, D. H. & Wiesel, T. N. Receptive fields, binocular interaction and functional architecture in the cat's visual cortex. J. Physiol. 160 , 106–154 (1962).
Reynaud, A., Masson, G. S. & Chavane, F. Dynamics of local input normalization result from balanced short- and long-range intracortical interactions in area V1. J. Neurosci. 32 , 12558–12569 (2012). This study proposes that horizontal propagation interacts with feedforward input through a common gain-control mechanism, implementing a dynamic surround suppression.
Ermentrout, G. B. & Kleinfeld, D. Traveling electrical waves in cortex: insights from phase dynamics and speculation on a computational role. Neuron 29 , 33–44 (2001).
Wilson, H. R. & Cowan, J. D. Excitatory and inhibitory interactions in localized populations of model neurons. Biophys. J. 12 , 1–24 (1972).
Helias, M. et al. Supercomputers ready for use as discovery machines for neuroscience. Front. Neuroinform. 6 , 26 (2012).
Bressloff, P. C. Spatiotemporal dynamics of continuum neural fields. J. Phys. A Math. Theor. 45 , 033001 (2011).
Ermentrout, B. Neural networks as spatio-temporal pattern-forming systems. Rep. Prog. Phys. 61 , 353–430 (1998).
Zheng, J., Lee, S. & Zhou, Z. J. A transient network of intrinsically bursting starburst cells underlies the generation of retinal waves. Nat. Neurosci. 9 , 363–371 (2006).
Ackman, J. B., Burbridge, T. J. & Crair, M. C. Retinal waves coordinate patterned activity throughout the developing visual system. Nature 490 , 219–225 (2012).
Braitenberg, V. & Schüz, A. Cortex: Statistics and Geometry of Neuronal Connectivity . (Springer, 1998).
Jeong, S.-O., Ko, T.-W. & Moon, H.-T. Time-delayed spatial patterns in a two-dimensional array of coupled oscillators. Phys. Rev. Lett. 89 , 154104 (2002).
Udeigwe, L. & Ermentrout, G. Waves and patterns on regular graphs. SIAM J. Appl. Dyn. Syst. 14 , 1102–1129 (2015).
Binzegger, T., Douglas, R. J. & Martin, K. A. C. A quantitative map of the circuit of cat primary visual cortex. J. Neurosci. 24 , 8441–8453 (2004).
Markov, N. T. et al. Weight consistency specifies regularities of macaque cortical networks. Cereb. Cortex 21 , 1254–1272 (2011).
Angelucci, A. et al. Circuits for local and global signal integration in primary visual cortex. J. Neurosci. 22 , 8633–8646 (2002).
Chen, Y. et al. The linearity and selectivity of neuronal responses in awake visual cortex. J. Vis. 9 , 12 (2009).
Destexhe, A. & Rudolph-Lilith, M. Neuronal Noise (Springer US, 2012).
Hô, N. & Destexhe, A. Synaptic background activity enhances the responsiveness of neocortical pyramidal neurons. J. Neurophysiol. 84 , 1488–1496 (2000).
Rudolph, M., Pospischil, M., Timofeev, I. & Destexhe, A. Inhibition determines membrane potential dynamics and controls action potential generation in awake and sleeping cat cortex. J. Neurosci. 27 , 5280–5290 (2007).
Somogyi, P. & Klausberger, T. Defined types of cortical interneurone structure space and spike timing in the hippocampus. J. Physiol. 562 , 9–26 (2005).
Fries, P., Reynolds, J. H., Rorie, A. E. & Desimone, R. Modulation of oscillatory neuronal synchronization by selective visual attention. Science 291 , 1560–1563 (2001).
Wang, X.-J. Neurophysiological and computational principles of cortical rhythms in cognition. Physiol. Rev. 90 , 1195–1268 (2010).
van Kerkoerle, T. et al. Alpha and gamma oscillations characterize feedback and feedforward processing in monkey visual cortex. Proc. Natl Acad. Sci. USA 111 , 14332–14341 (2014).
Pinto, D. J., Patrick, S. L., Huang, W. C. & Connors, B. W. Initiation, propagation, and termination of epileptiform activity in rodent neocortex in vitro involve distinct mechanisms. J. Neurosci. 25 , 8131–8140 (2005).
Trevelyan, A. J., Sussillo, D. & Yuste, R. Feedforward inhibition contributes to the control of epileptiform propagation speed. J. Neurosci. 27 , 3383–3387 (2007).
Klink, P. C., Dagnino, B., Gariel-Mathis, M.-A. & Roelfsema, P. R. Distinct feedforward and feedback effects of microstimulation in visual cortex reveal neural mechanisms of texture segregation. Neuron 95 , 209–220.e3 (2017).
Stettler, D. D., Das, A., Bennett, J. & Gilbert, C. D. Lateral connectivity and contextual interactions in macaque primary visual cortex. Neuron 36 , 739–750 (2002).
Osan, R. & Ermentrout, B. Two dimensional synaptically generated traveling waves in a theta-neuron neural network. Neurocomputing 38 , 789–795 (2001).
Veltz, R. & Faugeras, O. Stability of the stationary solutions of neural field equations with propagation delays. J. Math. Neurosci. 1 , 1 (2011).
Ermentrout, B. & Ko, T.-W. Delays and weakly coupled neuronal oscillators. Phil. Trans. A Math. Phys. Eng. Sci. 367 , 1097–1115 (2009).
Brunel, N. Dynamics of sparsely connected networks of excitatory and inhibitory spiking neurons. J. Comput. Neurosci. 8 , 183–208 (2000).
Bressloff, P. C. in Neural Fields: Theory and Applications (eds Coombes, S., beim Graben, P., Potthast, R. & Wright, J.) 235–268 (Springer, Berlin, Heidelberg, 2014).
Destexhe, A. & Contreras, D. Neuronal computations with stochastic network states. Science 314 , 85–90 (2006).
Morrison, A., Aertsen, A. & Diesmann, M. Spike-timing-dependent plasticity in balanced random networks. Neural Comput. 19 , 1437–1467 (2007).
Keane, A. & Gong, P. Propagating waves can explain irregular neural dynamics. J. Neurosci. 35 , 1591–1605 (2015).
Gong, P. & van Leeuwen, C. Distributed dynamical computation in neural circuits with propagating coherent activity patterns. PLoS Comput. Biol. 5 , e1000611 (2009).
Riesenhuber, M. & Poggio, T. Hierarchical models of object recognition in cortex. Nat. Neurosci. 2 , 1019–1025 (1999).
Blakemore, C. & Tobin, E. A. Lateral inhibition between orientation detectors in the cat's visual cortex. Exp. Brain Res. 15 , 439–440 (1972).
Gilbert, C. D. Adult cortical dynamics. Physiol. Rev. 78 , 467–485 (1998).
Allman, J., Miezin, F. & McGuinness, E. Stimulus specific responses from beyond the classical receptive field: neurophysiological mechanisms for local-global comparisons in visual neurons. Annu. Rev. Neurosci. 8 , 407–430 (1985).
Albright, T. D. & Stoner, G. R. Contextual influences on visual processing. Annu. Rev. Neurosci. 25 , 339–379 (2002).
Field, D. J., Hayes, A. & Hess, R. F. Contour integration by the human visual system: evidence for a local 'association field'. Vision Res. 33 , 173–193 (1993).
Polat, U. & Sagi, D. Lateral interactions between spatial channels: suppression and facilitation revealed by lateral masking experiments. Vision Res. 33 , 993–999 (1993).
Hess, R. F. & Dakin, S. C. Contour integration in the peripheral field. Vision Res. 39 , 947–959 (1999).
Ahmed, B. et al. Cortical dynamics subserving visual apparent motion. Cereb. Cortex 18 , 2796–2810 (2008).
Georges, S., Seriès, P., Frégnac, Y. & Lorenceau, J. Orientation dependent modulation of apparent speed: psychophysical evidence. Vision Res. 42 , 2757–2772 (2002).
Gerard-Mercier, F., Carelli, P. V., Pananceau, M., Troncoso, X. G. & Frégnac, Y. Synaptic correlates of low-level perception in V1. J. Neurosci. 36 , 3925–3942 (2016).
Carandini, M. et al. Do we know what the early visual system does? J. Neurosci. 25 , 10577–10597 (2005).
Olshausen, B. A. & Field, D. J. How close are we to understanding v1? Neural Comput. 17 , 1665–1699 (2005).
Sanes, J. N. & Donoghue, J. P. Oscillations in local field potentials of the primate motor cortex during voluntary movement. Proc. Natl Acad. Sci. USA 90 , 4470–4474 (1993).
Rubino, D., Robbins, K. A. & Hatsopoulos, N. G. Propagating waves mediate information transfer in the motor cortex. Nat. Neurosci. 9 , 1549–1557 (2006). This is one of the first studies to uncover travelling waves in the awake state and shows that these waves can carry task-relevant information during reaching behaviour in macaques.
Takahashi, K., Saleh, M., Penn, R. D. & Hatsopoulos, N. G. Propagating waves in human motor cortex. Front. Hum. Neurosci. 5 , 40 (2011).
Hatsopoulos, N. G., Olmedo, L. & Takahashi, K. in Motor Control (eds Danion, F. & Latash, M.) 159–176 (Oxford Univ. Press, 2010).
Riehle, A., Wirtssohn, S., Grün, S. & Brochier, T. Mapping the spatio-temporal structure of motor cortical LFP and spiking activities during reach-to-grasp movements. Front. Neural Circuits 7 , 48 (2013).
Kleiser, R., Seitz, R. J. & Krekelberg, B. Neural correlates of saccadic suppression in humans. Curr. Biol. 14 , 386–390 (2004).
Buzsáki, G. & Moser, E. I. Memory, navigation and theta rhythm in the hippocampal-entorhinal system. Nat. Neurosci. 16 , 130–138 (2013).
O'Keefe, J. & Recce, M. L. Phase relationship between hippocampal place units and the EEG theta rhythm. Hippocampus 3 , 317–330 (1993).
Colgin, L. L. Mechanisms and functions of theta rhythms. Annu. Rev. Neurosci. 36 , 295–312 (2013).
Bland, B. H., Anderson, P. & Ganes, T. Two generators of hippocampal theta activity in rabbits. Brain Res. 94 , 199–218 (1975).
Zhang, H. & Jacobs, J. Traveling theta waves in the human hippocampus. J. Neurosci. 35 , 12477–12487 (2015).
Jung, M. W., Wiener, S. I. & McNaughton, B. L. Comparison of spatial firing characteristics of units in dorsal and ventral hippocampus of the rat. J. Neurosci. 14 , 7347–7356 (1994).
Kjelstrup, K. B. et al. Finite scale of spatial representation in the hippocampus. Science 321 , 140–143 (2008).
Agarwal, G. et al. Spatially distributed local fields in the hippocampus encode rat position. Science 344 , 626–630 (2014).
Buzsáki, G., Horváth, Z., Urioste, R., Hetke, J. & Wise, K. High-frequency network oscillation in the hippocampus. Science 256 , 1025–1027 (1992).
Lee, A. K. & Wilson, M. A. Memory of sequential experience in the hippocampus during slow wave sleep. Neuron 36 , 1183–1194 (2002).
Patel, J., Schomburg, E. W., Berényi, A., Fujisawa, S. & Buzsáki, G. Local generation and propagation of ripples along the septotemporal axis of the hippocampus. J. Neurosci. 33 , 17029–17041 (2013).
Sejnowski, T. J. Storing covariance with nonlinearly interacting neurons. J. Math. Biol. 4 , 303–321 (1977).
Paulsen, O. & Sejnowski, T. J. Natural patterns of activity and long-term synaptic plasticity. Curr. Opin. Neurobiol. 10 , 172–179 (2000).
Huang, X., Elyada, Y. M., Bosking, W. H., Walker, T. & Fitzpatrick, D. Optogenetic assessment of horizontal interactions in primary visual cortex. J. Neurosci. 34 , 4976–4990 (2014).
Rankin, J. & Chavane, F. Neural field model to reconcile structure with function in primary visual cortex. PLoS Comput. Biol. 13 , e1005821 (2017).
Adamatzky, A., De Lacy Costello, B. & Asai, T. Reaction-Diffusion Computers . (Elsevier, 2005).
Adamatzky, A. & Durand-Lose, J. in Handbook of Natural Computing (eds Rozenberg, G., Bäck, T. & Kok, J. N.) 1949–1978 (Springer, Berlin, Heidelberg, 2012).
Poulet, J. F. A. & Petersen, C. C. H. Internal brain state regulates membrane potential synchrony in barrel cortex of behaving mice. Nature 454 , 881–885 (2008).
Ecker, A. S. et al. Decorrelated neuronal firing in cortical microcircuits. Science 327 , 584–587 (2010).
Perrard, S., Fort, E. & Couder, Y. Wave-based Turing machine: time reversal and information erasing. Phys. Rev. Lett. 117 , 094502 (2016).
Izhikevich, E. M. & Hoppensteadt, F. C. Polychronous wavefront computations. Int. J. Bifurcat. Chaos 19 , 1733–1739 (2009).
Chemla, S. et al. Improving voltage-sensitive dye imaging: with a little help from computational approaches. Neurophotonics 4 , 031215 (2017).
Han, X. et al. Millisecond-timescale optical control of neural dynamics in the nonhuman primate brain. Neuron 62 , 191–198 (2009).
Diester, I. et al. An optogenetic toolbox designed for primates. Nat. Neurosci. 14 , 387–397 (2011).
Download references
Acknowledgements
The authors thank Z. Davis, T. Bartol, G. Pao, A. Destexhe, Y. Frégnac and C. F. Stevens for helpful discussions and J. Ogawa for helpful discussions and help with illustrations. L.M. acknowledges support from the US National Institute of Mental Health (5T32MH020002-17). F.C. acknowledges support from Agence Nationale de la Recherche (ANR) projects BalaV1 (ANR-13-BSV4-0014-02) and Trajectory (ANR-15-CE37-0011-01). J.R. acknowledges support from the Fiona and Sanjay Jha Chair in Neuroscience at the Salk Institute. T.J.S. acknowledges support from Howard Hughes Medical Institute, Swartz Foundation and the Office of Naval Research (N000141210299).
Author information
Authors and affiliations.
Salk Institute for Biological Studies, La Jolla, CA, USA
Lyle Muller, John Reynolds & Terrence J. Sejnowski
Institut de Neurosciences de la Timone (INT), Centre National de la Recherche Scientifique (CNRS) and Aix-Marseille Université, Marseille, France
Frédéric Chavane
Division of Biological Sciences, University of California, La Jolla, CA, USA
Terrence J. Sejnowski
You can also search for this author in PubMed Google Scholar
Contributions
L.M., F.C., J.R. and T.J.S. researched data for the article, made substantial contributions to discussions of the content, wrote the article and reviewed and/or edited the manuscript before submission.
Corresponding author
Correspondence to Terrence J. Sejnowski .
Ethics declarations
Competing interests.
The authors declare no competing financial interests.
PowerPoint slides
Powerpoint slide for fig. 1, powerpoint slide for fig. 2, powerpoint slide for fig. 3, powerpoint slide for fig. 4, powerpoint slide for fig. 5, powerpoint slide for fig. 6, powerpoint slide for table 1.
The differences in phase (an amplitude-invariant measure of position in an oscillation cycle) between two (or more) oscillations.
A disturbance that travels through a physical medium that may be water, air or a neural network.
Patterns that can result from the summation of many individual waves. Depending on the properties of the medium, the pattern resulting from these interactions can differ greatly.
A scale between microscopic and macroscopic. In neuroscience, the mesoscopic scale describes single regions (such as cortical areas or subcortical nuclei) spanning millimetres to centimetres. Cortical networks at this scale can be imaged through recently developed recording technologies.
The scale of the whole brain; traditionally recorded with extracranial techniques (electroencephalography and magnetoencephalography) and more recently recorded with intracranial methods (electrocorticography).
(EEG). A neural recording technique in which electrodes are placed on the scalp, outside the skull (extracranial), that is of great use in studying the sensory and cognitive processes of normal human subjects.
(ECoG). A recording technique in which electrodes are placed directly on the cortical surface, offering both high spatial (up to 2 millimetres or greater) and high temporal resolution.
(LFP). The electric potential recorded in the extracellular space of the cortex. The LFP is thought to reflect the synaptic currents from neurons within a few hundred micrometres around the electrode.
(MEAs). One-dimensional or two-dimensional grids of electrodes, which offer the ability to sample local field potential and spiking activity at the mesoscopic scale.
(VSDs). Fluorescent dyes applied directly to the surface of the cortex that allow the subthreshold membrane potential of neural populations to be recorded. The resulting signals are linearly related to the average membrane potential of neurons at each point in the cortex. This technique captures neural activity over a large field of view with very high spatial (up to 20 micrometres) and temporal (up to 1 millisecond) resolution.
The large, 0.1–1.0 Hz rhythm of deep non-rapid-eye-movement sleep.
Passive transmission of an electric field through biological tissue. The fields can be created from a single source of neural activity and will appear as identical, highly synchronous waveforms across electrodes; a cause of spatial smoothing (blurring) in scalp electroencephalography.
A brief ( ∼ 1 second), biphasic waveform composed of a strong negative potential followed by a positive deflection. K-Complexes occur predominantly during stage 2 non-rapid-eye-movement sleep and are driven by transitions from cortical down to up states.
Thalamocortical 11–15 Hz oscillations prevalent in stage 2 non-rapid-eye-movement sleep. These oscillatory periods have long been associated with learning and memory, including sleep-dependent consolidation of long-term memory.
A group of interconnected, repeatedly co-activated neurons whose signature spike pattern is thought to collectively represent a specific sensory stimulus or memory.
Models of emergent collective behaviour in large ensembles. In these networks, individual units are characterized by a state (or phase) between 0 and 2π. Interactions among units are typically modelled as attractive, such that units with different states tend to synchronize depending on the coupling strength of the interaction.
A state of asynchronous, highly irregular firing in spiking network models. This state exhibits the low-correlated firing that is the hallmark of cortical dynamics under general conditions of excitatory and inhibitory balance.
An extension of the neural field model of Wilson and Cowan to include the effects of neural and synaptic noise.
In rodents, the long axis of the hippocampus, running from a dorsal, medial position to a ventral, lateral position; synonymous with septotemporal axis.
Models of chemical dynamics that take into account local reactions and diffusion across space. These reactions exhibit complex dynamics, including travelling waves and emergent patterns.
A property of a system whose dynamics remain the same when time is reversed. This feature implies important mathematical properties for the system under study.
Rights and permissions
Reprints and permissions
About this article
Cite this article.
Muller, L., Chavane, F., Reynolds, J. et al. Cortical travelling waves: mechanisms and computational principles. Nat Rev Neurosci 19 , 255–268 (2018). https://doi.org/10.1038/nrn.2018.20
Download citation
Published : 22 March 2018
Issue Date : May 2018
DOI : https://doi.org/10.1038/nrn.2018.20
Share this article
Anyone you share the following link with will be able to read this content:
Sorry, a shareable link is not currently available for this article.
Provided by the Springer Nature SharedIt content-sharing initiative
This article is cited by
Minute-scale oscillatory sequences in medial entorhinal cortex.
- Soledad Gonzalo Cogno
- Horst A. Obenhaus
- Edvard I. Moser
Nature (2024)
Mysterious ultraslow and ordered activity observed in the cortex
- Gilles Laurent
The direction of theta and alpha travelling waves modulates human memory processing
- Uma R. Mohan
- Honghui Zhang
- Joshua Jacobs
Nature Human Behaviour (2024)
Representing stimulus motion with waves in adaptive neural fields
- Zachary P Kilpatrick
Journal of Computational Neuroscience (2024)
Dynamics of neural fields with exponential temporal kernel
- Elham Shamsara
- Marius E. Yamakou
- Jürgen Jost
Theory in Biosciences (2024)
Quick links
- Explore articles by subject
- Guide to authors
- Editorial policies
Sign up for the Nature Briefing newsletter — what matters in science, free to your inbox daily.


Travelling Wave Tube (TWT)
A travelling wave tube is a high power amplifier used for the amplification of microwave signals up to a wide range. It is a special type of vacuum tube that offers an operating frequency ranging between 300 MHz to 50 GHz .
Travelling wave tubes are non-resonant structures that offer continuous interaction of applied RF field with the electron beam over the entire length of the tube. Due to this reason, it provides wider operating bandwidth.
Content: Travelling Wave Tube
Basic concept.
- Construction
Need of Slow-Wave Structure
- Applications
Travelling wave tubes are abbreviated as TWT . It is majorly used in the amplification of RF signals. Basically a travelling wave tube is nothing but an elongated vacuum tube that allows the movement of electron beam inside it by the action of applied RF input.
The movement of an electron inside the tube permits the amplification of applied RF input. As it offers amplification to a wide range of frequency thus is considered more advantageous for microwave applications than other tubes.
It offers average power gain of around 60 dB . The output power lies in the range of few watts to several megawatts.
A travelling wave tube is basically of two types one is helix type and the other is coupled cavity. Here in this section, we will discuss the detailed construction and working of a helical travelling wave tube.
Construction of Travelling Wave Tube
The figure here shows the constructional structure of a TWT:
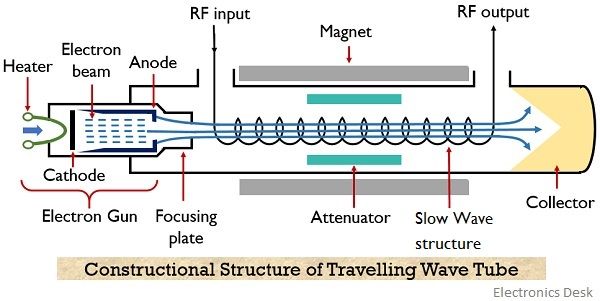
As we can see that the helical travelling wave tube consists of an electron gun and a slow-wave structure . The electron gun produces a narrow beam of the electron. A focusing plate is used that focuses the electron beam inside the tube.
A positive potential is provided to the coil (helix) with respect to the cathode terminal. While the collector is more positive than the coil (helix). In order to restrict beam spreading inside the tube. A dc magnetic field is applied between the travelling path by the help of magnets.
The signal which is needed to be amplified is provided at one of the ends of the helix, present adjacent to the electron gun. While the amplified signal is achieved at the opposite end of the helix.
In the figure, we can clearly see that attenuator is present along both the sides of the travelling wave tube. This is so because travelling wave amplifiers are high gain devices, so in case of poor load matching conditions, oscillations get build up inside the tube due to reflection.
Thus in order to restrict the generation of oscillations inside the tube attenuators are used.
Attenuators are basically formed by providing a metallic coating over the surface of the glass tube. Aquadag or Kanthal are majorly used for this.
It is to be noteworthy that a slow-wave structure is considered here, the reason is to maintain continuous interaction between the travelling wave and electron beam.
We know that the velocity of the electromagnetic wave is very much higher when compared with the phase velocity of the electron beam emitted by the electron gun.
Basically the RF wave applied at the input of TWT propagates with the speed of light (i.e., 3 * 10 8 m/s ). While the propagating velocity of the electron beam inside the tube is comparatively smaller than the velocity of RF wave.
If we try to somehow accelerate the velocity of the electron beam, then it can be accelerated only to a fraction of velocity of light. So it is better to reduce the velocity of the applied RF input in order to match the velocity of the electron beam.
Therefore, a slow-wave structure is used that causes a reduction in the phase velocity of the RF wave inside the TWT.
The slow-wave structures can be of different types like a single helix, double helix, zigzag line, corrugated, coupled-cavity or ring bar type etc.
A single helix slow-wave structure is formed by wounding a wire of element like tungsten and molybdenum in the form of a coil. The helical shape of the structure slows the velocity of the wave travelling along its axis to a fraction of about one-tenth of c.
This is so because due to the helical shape of the structure, the wave travels a much larger distance than the distance travelled by the beam inside the tube. So, in this way, the speed of wave propagation depends on the number of turns or diameter of the turns.
More specifically we can say that change in pitch can vary the speed of wave propagation inside the tube.
The equation given below shows the relation of phase velocity of the wave with the pitch of the helix:
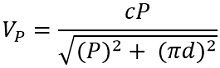
: c = velocity of light (3 * 10 8 m/s)
V P = phase velocity in m/s
P = pitch of helix in m
d = diameter of the helix in m
Therefore, this causes continuous interaction between the RF input wave and the electron beam as the velocity of propagation of the two is not highly different. As such interaction is the basis of working of TWT thus slow-wave structures are used.
Working of Travelling Wave Tube
Till now we have discussed the complete constructional structure of TWT. Let us now understand how the signal gets amplified while travelling inside the tube.
The applied RF signal produces an electric field inside the tube. Due to the applied positive half, the moving electron beam experiences accelerative force. However, the negative half of the input applies a de-accelerative force on the moving electrons.
This is said to be velocity modulation because the electrons of the beam are experiencing different velocity inside the tube.
However, the slowly travelling wave inside the tube exhibits continuous interaction with the electron beam.
Due to the continuous interaction, the electrons moving with high velocity transfer their energy to the wave inside the tube and thus slow down. So with the rise in the amplitude of the wave, the velocity of electrons reduces and this causes bunching of electrons inside the tube.
The growing amplitude of the wave resultantly causes more bunching of electrons while reaching the end from the beginning. Thereby causing further amplification of the RF wave inside the tube.
More specifically we can say that forward progression of the field along the axis of the tube gives rise to amplification of the RF wave. Thus at the end of the tube an amplified signal is achieved.
The positive potential provided at the other end causes collection of electron bunch at the collector.
The magnetic field inside the tube restricts the spreading of the beam as the electrons possess repulsive nature.
However, as the TWT is a bidirectional device . Therefore, the reflected signal causes oscillations inside the tube. But as we have already discussed earlier that the presence of attenuators reduces the generation of oscillations due to reflected backwave.
Sometimes despite using attenuators, internal impedance terminals are used that puts less lossy effects on the forward signal.
Applications of TWT
- Travelling wave tubes are highly used in continuous wave radar systems.
- These amplifying tubes also find application in broadband receivers for RF amplification.
- TWT’s are also used to get high power output in satellite transponders.
So from the above discussion, we can conclude that no resonant structure is present in the interaction space. Thus provides amplification up to a wide bandwidth operating range.
However, the input and output coupling arrangements must be considered carefully as they limit the operating range.
Related Terms:
- Transmission Lines
- IMPATT Diode
- Backward Wave Oscillator
Leave a Comment Cancel Reply
Your email address will not be published. Required fields are marked *
'He laughs. He cries': Caleb Williams' relatability, big arm go back to high school days
WASHINGTON – Caleb Williams was halfway across the country, but that didn’t stop him from attending his senior year statistics course at Gonzaga College High School.
“He would Zoom into my class every day from Norman, (Oklahoma),” said Terry Kernan, Gonzaga’s athletics director. “He would be in the football facility, or in his dorm room, or he’d be studying in the weight room. But he’d be there every day, just showing that commitment, where he could have easily just cashed it in and said, ‘I’m already at Oklahoma.‘ ”
These were abnormal circumstances for a student at Gonzaga, an all-boys Jesuit private school for grades 9-12 located roughly 10 blocks from the Capitol Building. These were also abnormal times. Like millions of Americans his age, Williams completed his senior year of high school (the 2020-21 academic year) virtually as the COVID-19 pandemic curtailed in-person learning. His senior football season was canceled.
Because he could Zoom into the classroom, Williams was able to enroll early at Oklahoma. And he was still able to complete his education at Gonzaga and, ultimately, graduate.
Doing both at the same time would have been a challenge had it not been for the pandemic, said Gonzaga’s special teams coordinator and manager of athletic communications Conrad Singh.
NFL DRAFT HUB: Latest NFL Draft mock drafts, news, live picks, grades and analysis.
“If there’s no pandemic, who knows if he wears a white tux?” said Singh, referencing the graduation attire worn by Gonzaga students. “I don’t know if that’s ever been said before. For him to be able to be at Oklahoma and here virtually, that’s unique. That’s not what Gonzaga does. If there’s no pandemic, that’s not what Gonzaga allows, so he would have been in a hard position to figure out ‘how can I be early enrolled?’”
It was a question Williams never had to answer. This preceded Williams’ lone season in Oklahoma. He subsequently transferred to Southern California, where he won the 2022 Heisman Trophy and solidified his standing as a top prospect widely expected to be taken first overall by the Chicago Bears in Thursday's NFL draft.
Williams, Singh said, displayed a different type of mental capacity to envision the future and create situations he wants. Then he goes after it.
“You believe in yourself and you create opportunity and choices,” Singh said. “That’s what it’s all about. And that’s what Caleb represents to a lot of people.”
Williams returned to Gonzaga for his No. 18 jersey retirement last May.
“He was right at home,” Singh said. “Firing the guys up, leading the fight song, he loves it here.”
On Thursday, Williams won’t be the only Gonzaga graduate to hear his name called by NFL Commissioner Roger Goodell. Penn State left tackle Olu Fashanu, who protected Williams’ blind side for two seasons at Gonzaga, is a projected first-round pick and will be one of the first offensive tackles off the board in a loaded class at the position.
A pair of former high-school teammates being taken in the same first round of the NFL draft isn’t unheard of. Bryant Westbrook (Lions) and Michael Booker (Falcons), for example, were teammates at El Camino High School in California and were selected six picks apart (fifth and 11th, respectively) in 1997.
That doesn't diminish the impact the selections of Williams and Fashanu will have on Gonzaga.
“Sometimes, it’s hard for people to have perspective on how powerful or unique something is,” Gonzaga head football coach Randy Trivers told USA TODAY Sports. “This is like an eclipse. This doesn’t happen.”
A 'relatable' QB and 'fresh mold of clay' on the line
Gonzaga beat out other schools in the D.C., Maryland, Virginia area and other prep powerhouses, such as IMG Academy in Florida, to secure Williams. The coaching staff scouted him heavily during when he was in eighth grade, current defensive coordinator and strength and conditioning coach Justin Young said. They knew he was special, Young said, but “there was just a different glow about him” upon his arrival on campus in 2017.
“I knew he had the potential to be the starting quarterback, which is rare for a freshman for us, at any position,” Trivers said.
The staff needed to see if he had another quality needed to be a freshman starter – the mental maturity that comes with the job. Throughout that first training camp, Williams passed each step. He digested the material in the meeting room and retained the information overnight. He proved he was tough enough during scrimmages.
“He’s relatable in the sense that he laughs. He cries. He’s human, just like us. He loves. He can have a conversation,” said Trivers, who will be in Detroit for the draft. “Sometimes, we see these people, we don't really know them, but we imagine what they are because they do these extraordinary things.”
Young was the offensive line coach during Williams’ freshman year and was responsible for developing a big-bodied sophomore who was still new to football: Fashanu. Now 6-foot-6 and 312 pounds, Fashanu went to Gonzaga as a basketball player who was young for his grade. He played on the freshman team before the varsity coaches brought him up the next year, Williams’ freshman campaign. Fashanu – who went on to win the 2023 Big Ten Offensive Lineman of the Year Award – didn’t play much. He spent plenty of time in the weight room with Young. Squats, explosion drills and flexibility exercises became his new language.
“He was like a fresh mold of clay. If we got him right, bending well, kick-stepping well, he was going to be well,” Young told USA TODAY Sports.
The Hail Mary: ‘Been riding that Caleb wave since’
Before being taken in the same first round, Williams and Fashanu were forever linked by Gonzaga’s 2018 Washington Catholic Athletic Conference championship – and “one of the best moments in Gonzaga history,” as Kernan put it.
Gonzaga entered the playoffs as the No. 4 seed and upset top-seeded St. John’s on the road with Fashanu, who became the starting left tackle that season, battling an injury. In the championship against DeMatha Catholic, there were three lead changes in the final 33 seconds, the last of which came on a Hail Mary fired more than 60 yards through the air by Williams and caught by receiver John Marshall as time expired.
The day before the championship, Gonzaga practiced that play on the same field going in the same direction.
“We were very poised to make that happen,” Singh said.
As Williams climbed the pocket as the final seconds ticked off, Fashanu kicked the pass rush out wide to give his quarterback enough space to unleash his prayer. Gonzaga defeated DeMatha, 46-43.
“We’ve been riding the Caleb wave since he threw that Hail Mary,” Young said.
Gonzaga lost in the WCAC semifinals in 2019, Fashanu's senior year and Williams' last season with the school due to the pandemic. More than four years later, they'll share the stage once again, this time in Detroit as NFL draftees.
“To have this No. 1 pick and another first-round pick, who played together, who are friends, who both developed under the system, who both have gone on and done great things, it would be incredible,” Kernan said.
For the two of them to have been teammates and champions has Gonzaga “buzzing” this week, Kernan said.
“They’re good friends to this day,” Young said. “They still talk. That’s just the brotherhood this school supplies.”
- Software Store
- Hot Topics:
TCL 50 XL 5G Android smartphone hits Metro by T-Mobile: Big features, small price

- By Brian Fagioli
- Published 2 days ago
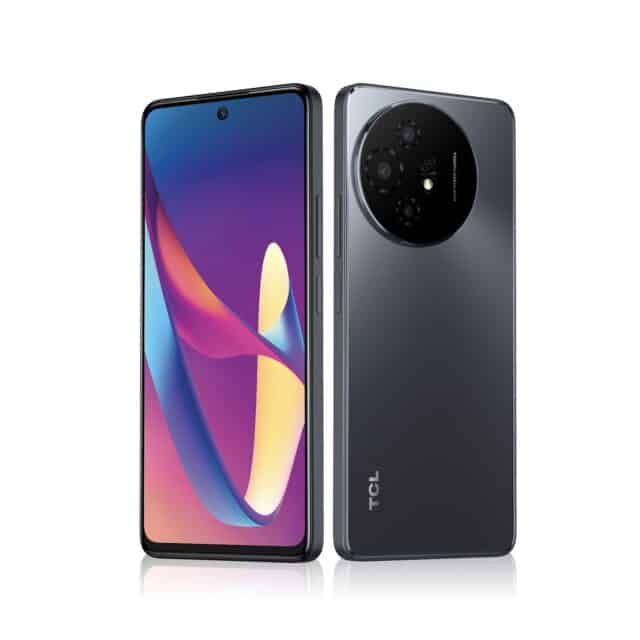
TCL Mobile is set to make waves in the budget smartphone market with the launch of the TCL 50 XL 5G, available at Metro by T-Mobile here now. As the inaugural device in the highly anticipated 50 Series, the TCL 50 XL 5G aims to bring high-end technology within reach of more consumers with its attractive price tag of just $159.
The TCL 50 XL 5G boasts a large 6.78-inch FHD+ display complemented by a smooth 120Hz refresh rate, ensuring vivid visuals and fluid motion for an immersive viewing experience. This smartphone is not just about the display; it also features dual speakers enhanced with DTS sound technology, offering a superior audio experience for both headphone users and open-air listening.
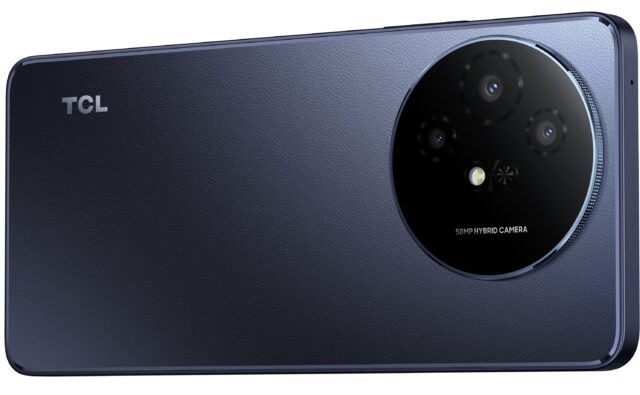
The phone's large battery life supports extensive use, making it a suitable companion for users who consume and create content without the worry of frequent recharges. Additionally, the TCL 50 XL 5G includes a powerful 50MP triple camera array, catering to content creators looking for a capable yet affordable device to capture high-quality photos and videos.
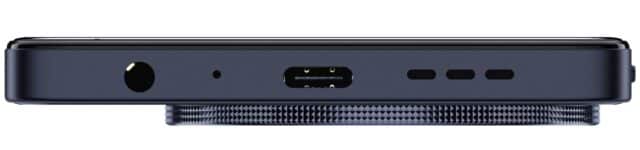
The combination of advanced features at a low cost highlights TCL's commitment to accessibility, ensuring that more users can enjoy the benefits of 5G connectivity and multimedia capabilities without breaking the bank. The TCL 50 XL 5G stands out as a multimedia powerhouse, promising to deliver performance and value in one package.
Recent Headlines
Human risk management automation can help beat burnout, vivaldi 6.7 debuts memory saver performance booster, expands feed reader capabilities, best windows apps this week, addressing digital transformation needs in the public sector [q&a], the psychological impact of phishing attacks on your employees, canonical releases ubuntu linux 24.04 lts 'noble numbat', new tool lets enterprises build their own secure gen ai chatbots, most commented stories, say goodbye to microsoft windows 11 and hello to nitrux linux 3.4.0 'pl', the stunning windows 13 -- yes, 13 -- is the microsoft operating system we want, microsoft 'improves' windows 11 by bringing ads to the start menu in the us, microsoft is up to its old tricks yet again -- windows 10 users harassed with full-screen windows 11 upgrade warnings, windows 11 slammed for its 'comically bad' performance even on high-end hardware, outrageous: microsoft to charge $61 for windows 10 updates -- consider switching to linux, microsoft releases preview version of office 2024 for windows and macos -- download it now, easter giveaway get a licensed copy of 'videoproc converter for windows/mac' (worth $78.90) for free.
© 1998-2024 BetaNews, Inc. All Rights Reserved. Privacy Policy - Cookie Policy .
Mountain View, CA

Mountain View
Around the Globe
Hurricane tracker.
Severe Weather
Radar & Maps
News & features, winter center, news / weather news, indian voters battle extreme temperatures as intense heat wave hits region.
By Helen Regan and Esha Mitra, CNN
Published Apr 26, 2024 3:50 AM PDT | Updated Apr 26, 2024 3:50 AM PDT

A taxi driver drinks water during a heat wave in Kolkata, India, on April 21. (Photo credit: Sudipta Das/NurPhoto/Getty Images via CNN Newsource)
New Delhi (CNN) — Indian voters are battling sweltering conditions to take part in the world’s biggest election as a severe heat wave hits parts of the country and authorities forecast a hotter-than-normal summer for the South Asian nation.
The India Meteorological Department (IMD) said a heat wave will affect parts of south and east India until the end of the week, including four states that are voting on Friday .
Parts of West Bengal, Bihar, Uttar Pradesh and Karnataka are among 13 states and union territories voting in the second phase of India’s mammoth elections , with temperatures forecast to exceed 40 degrees Celsius (104 degrees Fahrenheit) in some areas.
On Thursday, Baripada in the eastern state of Odisha hit 43.6 C (110.4 F) and Telangana’s Khammam in the south reached 43.4 C (110.1 F), according to the IMD, which warned last month that India would likely see stronger and longer heat waves this year due to above-normal temperatures.
Gandhi Ray, a farmer in his 60s from eastern Bihar state, said he lives in a small hut in the forest, and will walk to a nearby village to vote.
Temperatures above 41 C (105 F) are forecast every day until May 1 in his hometown of Banka district, according to the IMD.
“It’s important for me to vote but definitely every day this heat is getting worse and worse,” he told CNN. “I work outdoors mostly so I am used to it but as I get older it becomes harder to cope. Now my kids have taken over most of the work.”

High temperatures have raised concern this election cycle, as campaigns marked by outdoor political rallies draw thousands of people under the baking sun. The issue was underscored Wednesday, when one lawmaker collapsed from the heat while addressing supporters in western Maharashtra state.
The Election Commission, National Disaster Management Authority and IMD formed a task force to minimize the impact of heat waves ahead of polling days and Prime Minister Narendra Modi chaired a meeting earlier this month to review the country’s preparedness for the hot season.
The Election Commission has released guidelines for staying cool at polling stations, including drinking water and carrying an umbrella, and warned against leaving children or pets in parked cars.
And in Bihar, election officials have extended voting hours at some polling stations “in view (of the) prevailing heat wave.”
Ray said the heat isn’t going to stop him from voting on Friday.
“This is the one right we have so of course I will vote, everyone should vote for whomever they want to represent them,” he said.
“Of course it would be good if the election took place in a cooler time but whether I go to vote or not, I am still going to feel hot so that’s not going to stop me.”
Despite the heat warnings, the Election Commision said there are “no major concerns for heat waves” during Friday’s polling and weather forecasts indicate “normal conditions” for the constituencies voting.
Climate politics
India, the world’s most populous nation with 1.4 billion people, often experiences heat waves during the summer months of May and June. But in recent years, they have arrived earlier and become more prolonged, with scientists linking some of these longer and more intense heat waves to the climate crisis.
In 2022, a heat wave that killed 90 people across India and Pakistan was made 30 times more likely because of climate change, the World Weather Attribution initiative found.
Last year successive heat waves hit India again, closing schools, damaging crops and putting pressure on energy supplies. In June alone, temperatures in some parts of the country soared to 47 C (116 F), killing at least 44 people and sickening hundreds with heat-related illnesses.

A man carries ice on his bicycle in Mumbai, India on Sunday, April 14. (Photo credit: Noemi Cassanelli/CNN via CNN Newsource)
The human-caused climate crisis is already threatening India’s development goals and putting millions of people at risk in a nation where more than 50% of the workforce is employed in agriculture, studies have found. By 2050, India will be among the places where temperatures have passed survivability limits, according to climate experts.
But analysts say climate has not featured as a major issue this election, despite being mentioned in the election manifestos of the two main parties — the ruling Bharatiya Janata Party (BJP) and the Indian National Congress.
“Climate impacts do shape voter demands — though this tends to filter through as anxieties about livelihood and continued welfare support, rather than in a neatly defined area of politics labeled ‘climate’,” said Aditya Valiathan Pillai , fellow and coordinator for adaptation and resilience at the New Delhi-based Sustainable Futures Collaborative, in a recent op-ed for CNN.
“You can see it in farmers asking for loan waivers and irrigation facilities after years of drought, in urban families demanding reduced electricity prices to offset cooling bills and in calls for more penetrating social welfare.”

Regional impact
Extreme heat has already had an impact across the region this year with little respite from merciless heat and humidity for hundreds of millions of people living in areas most vulnerable to climate change.
Neighboring Bangladesh is sweltering through a heat wave this week with prolonged temperatures above 40 C in many districts and no relief during record hot nights, according to climatologist Maximiliano Herrera. The government declared a “heat alert” across the country on Thursday, in place for 72 hours.
Extreme temperatures are also soaring across Southeast Asia , with dozens of heatstroke deaths report by local media in Thailand, hundreds of schools closed in the Philippines, and droughts drying up rice fields and rivers in Vietnam’s Mekong Delta “rice bowl” region.
The weeks-long heat wave in Vietnam has forced three provinces to declare a state of emergency as salt seeps into fresh water sources, limiting access to drinking water for more than 70,000 households, according to Save the Children.
A report released Tuesday by the World Meteorological Organization found that Asia remained the world’s most disaster-affected region in 2023 and the region is heating up faster than the global average.

The-CNN-Wire™ & © 2024 Cable News Network, Inc., a Warner Bros. Discovery Company. All rights reserved.
Weather News

LIVE: Several tornadoes, large hail reported Thursday

How to recognize a 'radar-confirmed tornado'
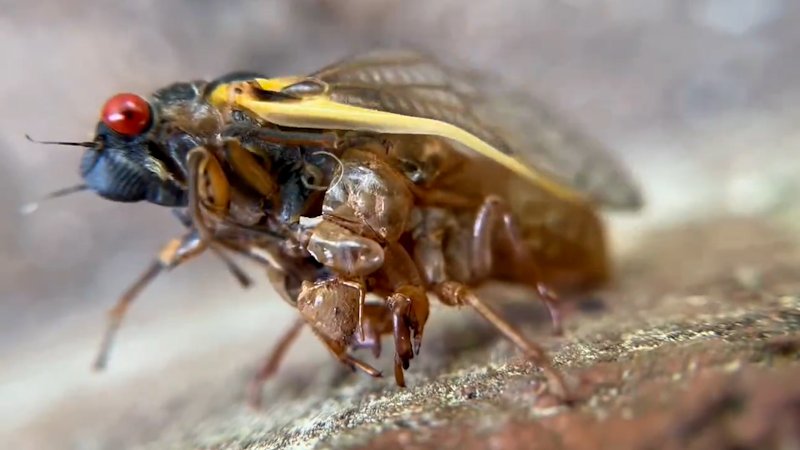
Two broods of cicadas begin to emerge up and down the East Coast
Top Stories
Trending Today
Accuweather early, accuweather prime, forecast victories.
LATEST ENTRY
Thursday’s storms seen from space
21 minutes ago

Dangerous US severe weather outbreak to peak on Friday, Saturday
16 minutes ago

This map may save lives when there's a tornado threat

Weather Forecasts
Warmth to surge over much of Northeast; 1st 90s F in some areas
18 hours ago

911 call frenzy as cicadas invade South Carolina towns
22 hours ago

Featured Stories
10 types of tornadoes that occur in the US

Overrun Japanese town erects barrier to block photos of Mount Fuji

Indian voters battle extreme temperatures amid intense heat wave
2 hours ago

A lake in Mexico’s ‘magical town’ is disappearing

More than 100 prisoners flee after rainfall destroys prison in Nigeria
3 hours ago

We have updated our Privacy Policy and Cookie Policy .
Get AccuWeather alerts as they happen with our browser notifications.
Notifications Enabled
Thanks! We’ll keep you informed.

IMAGES
VIDEO
COMMENTS
Describing a Wave. A wave can be described as a disturbance in a medium that travels transferring momentum and energy without any net motion of the medium. A wave in which the positions of maximum and minimum amplitude travel through the medium is known as a travelling wave. To better understand a wave, let us think of the disturbance caused ...
Waves can be "traveling" or "standing," and we will start with the traveling kind, since they are the ones that most clearly exhibit the characteristics typically associated with wave motion. A traveling wave in a medium is a disturbance of the medium that propagates through it, in a definite direction and with a definite velocity. By a ...
Figure 16.2 An ocean wave is probably the first picture that comes to mind when you hear the word "wave." Although this breaking wave, and ocean waves in general, have apparent similarities to the basic wave characteristics we will discuss, the mechanisms driving ocean waves are highly complex and beyond the scope of this chapter.
A wave is a disturbance that propagates, or moves from the place it was created. There are three basic types of waves: mechanical waves, electromagnetic waves, and matter waves. Basic mechanical waves are governed by Newton's laws and require a medium. A medium is the substance a mechanical waves propagates through, and the medium produces an ...
A wave is a repeating disturbance that travels through matter or space transferring only energy. Below is a model of a wave. A wave's crest is its highest point, and its trough is its lowest point. A wave's amplitude is the maximum distance (positive or negative) a wave reaches from its rest position. Wavelength is the distance between the ...
A wave is just the phenomenon of oscillation of energy, using various properties of a medium such as physical, electro-magnetic, etc. For example, sound waves and light waves are both the carriers of energy, but a sound wave propagates through pressure variations, whereas a light wave travels by making use of electro-magnetic phenomena, which ...
A wave is a disturbance that propagates, or moves from the place it was created. There are three basic types of waves: mechanical waves, electromagnetic waves, and matter waves. Basic mechanical waves are governed by Newton's laws and require a medium. A medium is the substance a mechanical waves propagates through, and the medium produces an ...
Longitudinal waves form when the particles of the medium vibrate back and forth in the same direction of the traveling wave. The wave can be visualized as compressions and expansions travelling along the medium. The distance between adjacent compressions is the wavelength. Figure 2: Parts of a longitudinal wave.
ω t = A cos. . ( k x − ω t), which is a wave traveling from x = 0 x = 0 to x = L x = L. The crucial point is that the two standing waves out of which the traveling wave is built are 90∘ 90 ∘ out of phase with one another both in time and in space. They get large at different points in space and also at different times and the ...
A wave is a repeating disturbance that travels through matter or space transferring only energy. Below is a model of a wave. A wave's crest is its highest point, and its trough is its lowest point. A wave's amplitude is the maximum distance (positive or negative) a wave reaches from its rest position. Wavelength is the distance between the ...
However, as mentioned in the text feature on surfing, actual ocean waves are more complex than this simplified example. Figure 13.7 The wave has a wavelength ... Shear or transverse waves cannot travel through a liquid and are not transmitted through Earth's core. In contrast, compression or longitudinal waves can pass through a liquid and ...
Traveling Waves vs. Standing Waves. A mechanical wave is a disturbance that is created by a vibrating object and subsequently travels through a medium from one location to another, transporting energy as it moves. The mechanism by which a mechanical wave propagates itself through a medium involves particle interaction; one particle applies a ...
Waves are cool. The more we learn about waves, the more we learn about a lot of things in physics. Everything from earthquakes to music! Ropes can tell us a ...
Wave model • describes behavior common to all waves • Traveling wave: organized (collective) disturbance traveling at a well-defined speed, v • 3 types: (i) Mechanical (within a material medium) e.g. sound in air, ripples on water (ii) Electromagnetic (light): oscillation of field, can travel in vacuum
MIT 8.03SC Physics III: Vibrations and Waves, Fall 2016View the complete course: https://ocw.mit.edu/8-03SCF16Instructor: Yen-Jie LeeProf. Lee introduces the...
A travelling wave is a continuous disturbance in a medium characterized by repeating oscillations. For example: ... Sound waves are longitudinal waves. 4.3 - Wave characteristics. Wavefronts and rays; Wavefronts: Lines joining points which vibrate in phase. Can be straight lines or curves.
And so the speed of features of the wave along the x-direction is Q: Do the units match on both sides? Another way to make waves travel There is another way we can start with the equation for a stationary wave and derive equations for travelling waves. ... Yes, it's slightly annoying that a wave travelling in the POSITIVE x-direction has an ...
A lecture covering wave characteristics and definitions. Types of waves, what makes up different types of waves, and the wave speed equation.
A traveling wave is a disturbance that travels through a medium. Consider the waves made by fans at a soccer game, as in Figure 14.1.1 14.1. 1. The fans can be thought of as the medium through which the wave propagates. The elements of the medium may oscillate about an equilibrium position (the fans move a short distance up and down), but they ...
Travelling waves are the kind of waves where the position of the particles shifts as the wave advances. The wave's maximum and minimum amplitudes pass across the medium, and its constituent particles oscillate in accordance with the wave's progression. Progressive waves are another name for moving waves.
Taken together, these results indicate that stimulus-evoked travelling waves are a general and important feature of dynamics in the visual system that can affect neuronal processing.
A traveling-wave tube ( TWT, pronounced "twit" [1]) or traveling-wave tube amplifier ( TWTA, pronounced "tweeta") is a specialized vacuum tube that is used in electronics to amplify radio frequency (RF) signals in the microwave range. [2] It was invented by Andrei Haeff around 1933 as a graduate student at Caltech, and its present form was ...
A travelling wave tube is a high power amplifier used for the amplification of microwave signals up to a wide range. It is a special type of vacuum tube that offers an operating frequency ranging between 300 MHz to 50 GHz.. Travelling wave tubes are non-resonant structures that offer continuous interaction of applied RF field with the electron beam over the entire length of the tube.
"We've been riding the Caleb wave since he threw that Hail Mary," Young said. Gonzaga lost in the WCAC semifinals in 2019, Fashanu's senior year and Williams' last season with the school due ...
TCL Mobile is set to make waves in the budget smartphone market with the launch of the TCL 50 XL 5G, available at Metro by T-Mobile here now. As the inaugural device in the highly anticipated 50 ...
Indian voters are battling sweltering conditions to take part in the world's biggest election as a severe heat wave hits parts of the country. Go Back LIVE: Severe storms threaten central U.S ...