21.4 Transmutation and Nuclear Energy
Learning objectives.
By the end of this section, you will be able to:
- Describe the synthesis of transuranium nuclides
- Explain nuclear fission and fusion processes
- Relate the concepts of critical mass and nuclear chain reactions
- Summarize basic requirements for nuclear fission and fusion reactors
After the discovery of radioactivity, the field of nuclear chemistry was created and developed rapidly during the early twentieth century. A slew of new discoveries in the 1930s and 1940s, along with World War II, combined to usher in the Nuclear Age in the mid-twentieth century. Scientists learned how to create new substances, and certain isotopes of certain elements were found to possess the capacity to produce unprecedented amounts of energy, with the potential to cause tremendous damage during war, as well as produce enormous amounts of power for society’s needs during peace.

Synthesis of Nuclides
Nuclear transmutation is the conversion of one nuclide into another. It can occur by the radioactive decay of a nucleus, or the reaction of a nucleus with another particle. The first manmade nucleus was produced in Ernest Rutherford’s laboratory in 1919 by a transmutation reaction, the bombardment of one type of nuclei with other nuclei or with neutrons. Rutherford bombarded nitrogen atoms with high-speed α particles from a natural radioactive isotope of radium and observed protons resulting from the reaction:
The 8 17 O 8 17 O and 1 1 H 1 1 H nuclei that are produced are stable, so no further (nuclear) changes occur.
To reach the kinetic energies necessary to produce transmutation reactions, devices called particle accelerators are used. These devices use magnetic and electric fields to increase the speeds of nuclear particles. In all accelerators, the particles move in a vacuum to avoid collisions with gas molecules. When neutrons are required for transmutation reactions, they are usually obtained from radioactive decay reactions or from various nuclear reactions occurring in nuclear reactors. The Chemistry in Everyday Life feature that follows discusses a famous particle accelerator that made worldwide news.
Chemistry in Everyday Life
Cern particle accelerator.
Located near Geneva, the CERN (“Conseil Européen pour la Recherche Nucléaire,” or European Council for Nuclear Research) Laboratory is the world’s premier center for the investigations of the fundamental particles that make up matter. It contains the 27-kilometer (17 mile) long, circular Large Hadron Collider (LHC), the largest particle accelerator in the world ( Figure 21.13 ). In the LHC, particles are boosted to high energies and are then made to collide with each other or with stationary targets at nearly the speed of light. Superconducting electromagnets are used to produce a strong magnetic field that guides the particles around the ring. Specialized, purpose-built detectors observe and record the results of these collisions, which are then analyzed by CERN scientists using powerful computers.
In 2012, CERN announced that experiments at the LHC showed the first observations of the Higgs boson, an elementary particle that helps explain the origin of mass in fundamental particles. This long-anticipated discovery made worldwide news and resulted in the awarding of the 2013 Nobel Prize in Physics to François Englert and Peter Higgs, who had predicted the existence of this particle almost 50 years previously.
Link to Learning
Famous physicist Brian Cox talks about his work on the Large Hadron Collider at CERN, providing an entertaining and engaging tour of this massive project and the physics behind it.
View a short video from CERN, describing the basics of how its particle accelerators work.
Prior to 1940, the heaviest-known element was uranium, whose atomic number is 92. Now, many artificial elements have been synthesized and isolated, including several on such a large scale that they have had a profound effect on society. One of these—element 93, neptunium (Np)—was first made in 1940 by McMillan and Abelson by bombarding uranium-238 with neutrons. The reaction creates unstable uranium-239, with a half-life of 23.5 minutes, which then decays into neptunium-239. Neptunium-239 is also radioactive, with a half-life of 2.36 days, and it decays into plutonium-239. The nuclear reactions are:
Plutonium is now mostly formed in nuclear reactors as a byproduct during the fission of U-235. Additional neutrons are released during this fission process (see the next section), some of which combine with U-238 nuclei to form uranium-239; this undergoes β decay to form neptunium-239, which in turn undergoes β decay to form plutonium-239 as illustrated in the preceding three equations. These processes are summarized in the equation:
Heavier isotopes of plutonium—Pu-240, Pu-241, and Pu-242—are also produced when lighter plutonium nuclei capture neutrons. Some of this highly radioactive plutonium is used to produce military weapons, and the rest presents a serious storage problem because they have half-lives from thousands to hundreds of thousands of years.
Although they have not been prepared in the same quantity as plutonium, many other synthetic nuclei have been produced. Nuclear medicine has developed from the ability to convert atoms of one type into other types of atoms. Radioactive isotopes of several dozen elements are currently used for medical applications. The radiation produced by their decay is used to image or treat various organs or portions of the body, among other uses.
The elements beyond element 92 (uranium) are called transuranium elements . As of this writing, 22 transuranium elements have been produced and officially recognized by IUPAC; several other elements have formation claims that are waiting for approval. Some of these elements are shown in Table 21.3 .
Nuclear Fission
Many heavier elements with smaller binding energies per nucleon can decompose into more stable elements that have intermediate mass numbers and larger binding energies per nucleon—that is, mass numbers and binding energies per nucleon that are closer to the “peak” of the binding energy graph near 56 (see Figure 21.3 ). Sometimes neutrons are also produced. This decomposition is called fission , the breaking of a large nucleus into smaller pieces. The breaking is rather random with the formation of a large number of different products. Fission usually does not occur naturally, but is induced by bombardment with neutrons. The first reported nuclear fission occurred in 1939 when three German scientists, Lise Meitner, Otto Hahn, and Fritz Strassman, bombarded uranium-235 atoms with slow-moving neutrons that split the U-238 nuclei into smaller fragments that consisted of several neutrons and elements near the middle of the periodic table. Since then, fission has been observed in many other isotopes, including most actinide isotopes that have an odd number of neutrons. A typical nuclear fission reaction is shown in Figure 21.14 .
Among the products of Meitner, Hahn, and Strassman’s fission reaction were barium, krypton, lanthanum, and cerium, all of which have nuclei that are more stable than uranium-235. Since then, hundreds of different isotopes have been observed among the products of fissionable substances. A few of the many reactions that occur for U-235, and a graph showing the distribution of its fission products and their yields, are shown in Figure 21.15 . Similar fission reactions have been observed with other uranium isotopes, as well as with a variety of other isotopes such as those of plutonium.
View this link to see a simulation of nuclear fission.
A tremendous amount of energy is produced by the fission of heavy elements. For instance, when one mole of U-235 undergoes fission, the products weigh about 0.2 grams less than the reactants; this “lost” mass is converted into a very large amount of energy, about 1.8 × × 10 10 kJ per mole of U-235. Nuclear fission reactions produce incredibly large amounts of energy compared to chemical reactions. The fission of 1 kilogram of uranium-235, for example, produces about 2.5 million times as much energy as is produced by burning 1 kilogram of coal.
As described earlier, when undergoing fission U-235 produces two “medium-sized” nuclei, and two or three neutrons. These neutrons may then cause the fission of other uranium-235 atoms, which in turn provide more neutrons that can cause fission of even more nuclei, and so on. If this occurs, we have a nuclear chain reaction (see Figure 21.16 ). On the other hand, if too many neutrons escape the bulk material without interacting with a nucleus, then no chain reaction will occur.
Material that can sustain a nuclear fission chain reaction is said to be fissile or fissionable . (Technically, fissile material can undergo fission with neutrons of any energy, whereas fissionable material requires high-energy neutrons.) Nuclear fission becomes self-sustaining when the number of neutrons produced by fission equals or exceeds the number of neutrons absorbed by splitting nuclei plus the number that escape into the surroundings. The amount of a fissionable material that will support a self-sustaining chain reaction is a critical mass . An amount of fissionable material that cannot sustain a chain reaction is a subcritical mass . An amount of material in which there is an increasing rate of fission is known as a supercritical mass . The critical mass depends on the type of material: its purity, the temperature, the shape of the sample, and how the neutron reactions are controlled ( Figure 21.17 ).
An atomic bomb ( Figure 21.18 ) contains several pounds of fissionable material, 92 235 U 92 235 U or 94 239 Pu , 94 239 Pu , a source of neutrons, and an explosive device for compressing it quickly into a small volume. When fissionable material is in small pieces, the proportion of neutrons that escape through the relatively large surface area is great, and a chain reaction does not take place. When the small pieces of fissionable material are brought together quickly to form a body with a mass larger than the critical mass, the relative number of escaping neutrons decreases, and a chain reaction and explosion result.
Fission Reactors
Chain reactions of fissionable materials can be controlled and sustained without an explosion in a nuclear reactor ( Figure 21.19 ). Any nuclear reactor that produces power via the fission of uranium or plutonium by bombardment with neutrons must have at least five components: nuclear fuel consisting of fissionable material, a nuclear moderator, reactor coolant, control rods, and a shield and containment system. We will discuss these components in greater detail later in the section. The reactor works by separating the fissionable nuclear material such that a critical mass cannot be formed, controlling both the flux and absorption of neutrons to allow shutting down the fission reactions. In a nuclear reactor used for the production of electricity, the energy released by fission reactions is trapped as thermal energy and used to boil water and produce steam. The steam is used to turn a turbine, which powers a generator for the production of electricity.
Nuclear Fuels
Nuclear fuel consists of a fissionable isotope, such as uranium-235, which must be present in sufficient quantity to provide a self-sustaining chain reaction. In the United States, uranium ores contain from 0.05–0.3% of the uranium oxide U 3 O 8 ; the uranium in the ore is about 99.3% nonfissionable U-238 with only 0.7% fissionable U-235. Nuclear reactors require a fuel with a higher concentration of U-235 than is found in nature; it is normally enriched to have about 5% of uranium mass as U-235. At this concentration, it is not possible to achieve the supercritical mass necessary for a nuclear explosion. Uranium can be enriched by gaseous diffusion (the only method currently used in the US), using a gas centrifuge, or by laser separation.
In the gaseous diffusion enrichment plant where U-235 fuel is prepared, UF 6 (uranium hexafluoride) gas at low pressure moves through barriers that have holes just barely large enough for UF 6 to pass through. The slightly lighter 235 UF 6 molecules diffuse through the barrier slightly faster than the heavier 238 UF 6 molecules. This process is repeated through hundreds of barriers, gradually increasing the concentration of 235 UF 6 to the level needed by the nuclear reactor. The basis for this process, Graham’s law, is described in the chapter on gases. The enriched UF 6 gas is collected, cooled until it solidifies, and then taken to a fabrication facility where it is made into fuel assemblies. Each fuel assembly consists of fuel rods that contain many thimble-sized, ceramic-encased, enriched uranium (usually UO 2 ) fuel pellets. Modern nuclear reactors may contain as many as 10 million fuel pellets. The amount of energy in each of these pellets is equal to that in almost a ton of coal or 150 gallons of oil.
Nuclear Moderators
Neutrons produced by nuclear reactions move too fast to cause fission (refer back to Figure 21.17 ). They must first be slowed to be absorbed by the fuel and produce additional nuclear reactions. A nuclear moderator is a substance that slows the neutrons to a speed that is low enough to cause fission. Early reactors used high-purity graphite as a moderator. Modern reactors in the US exclusively use heavy water ( 1 2 H 2 O ) ( 1 2 H 2 O ) or light water (ordinary H 2 O), whereas some reactors in other countries use other materials, such as carbon dioxide, beryllium, or graphite.
Reactor Coolants
A nuclear reactor coolant is used to carry the heat produced by the fission reaction to an external boiler and turbine, where it is transformed into electricity. Two overlapping coolant loops are often used; this counteracts the transfer of radioactivity from the reactor to the primary coolant loop. All nuclear power plants in the US use water as a coolant. Other coolants include molten sodium, lead, a lead-bismuth mixture, or molten salts.
Control Rods
Nuclear reactors use control rods ( Figure 21.20 ) to control the fission rate of the nuclear fuel by adjusting the number of slow neutrons present to keep the rate of the chain reaction at a safe level. Control rods are made of boron, cadmium, hafnium, or other elements that are able to absorb neutrons. Boron-10, for example, absorbs neutrons by a reaction that produces lithium-7 and alpha particles:
When control rod assemblies are inserted into the fuel element in the reactor core, they absorb a larger fraction of the slow neutrons, thereby slowing the rate of the fission reaction and decreasing the power produced. Conversely, if the control rods are removed, fewer neutrons are absorbed, and the fission rate and energy production increase. In an emergency, the chain reaction can be shut down by fully inserting all of the control rods into the nuclear core between the fuel rods.
Shield and Containment System
During its operation, a nuclear reactor produces neutrons and other radiation. Even when shut down, the decay products are radioactive. In addition, an operating reactor is thermally very hot, and high pressures result from the circulation of water or another coolant through it. Thus, a reactor must withstand high temperatures and pressures, and must protect operating personnel from the radiation. Reactors are equipped with a containment system (or shield) that consists of three parts:
- The reactor vessel, a steel shell that is 3–20-centimeters thick and, with the moderator, absorbs much of the radiation produced by the reactor
- A main shield of 1–3 meters of high-density concrete
- A personnel shield of lighter materials that protects operators from γ rays and X-rays
In addition, reactors are often covered with a steel or concrete dome that is designed to contain any radioactive materials might be released by a reactor accident.
Click here to watch a 3-minute video from the Nuclear Energy Institute on how nuclear reactors work.
Nuclear power plants are designed in such a way that they cannot form a supercritical mass of fissionable material and therefore cannot create a nuclear explosion. But as history has shown, failures of systems and safeguards can cause catastrophic accidents, including chemical explosions and nuclear meltdowns (damage to the reactor core from overheating). The following Chemistry in Everyday Life feature explores three infamous meltdown incidents.
Nuclear Accidents
The importance of cooling and containment are amply illustrated by three major accidents that occurred with the nuclear reactors at nuclear power generating stations in the United States (Three Mile Island), the former Soviet Union (Chernobyl), and Japan (Fukushima).
In March 1979, the cooling system of the Unit 2 reactor at Three Mile Island Nuclear Generating Station in Pennsylvania failed, and the cooling water spilled from the reactor onto the floor of the containment building. After the pumps stopped, the reactors overheated due to the high radioactive decay heat produced in the first few days after the nuclear reactor shut down. The temperature of the core climbed to at least 2200 °C, and the upper portion of the core began to melt. In addition, the zirconium alloy cladding of the fuel rods began to react with steam and produced hydrogen:
The hydrogen accumulated in the confinement building, and it was feared that there was danger of an explosion of the mixture of hydrogen and air in the building. Consequently, hydrogen gas and radioactive gases (primarily krypton and xenon) were vented from the building. Within a week, cooling water circulation was restored and the core began to cool. The plant was closed for nearly 10 years during the cleanup process.
Although zero discharge of radioactive material is desirable, the discharge of radioactive krypton and xenon, such as occurred at the Three Mile Island plant, is among the most tolerable. These gases readily disperse in the atmosphere and thus do not produce highly radioactive areas. Moreover, they are noble gases and are not incorporated into plant and animal matter in the food chain. Effectively none of the heavy elements of the core of the reactor were released into the environment, and no cleanup of the area outside of the containment building was necessary ( Figure 21.21 ).
Another major nuclear accident involving a reactor occurred in April 1986, at the Chernobyl Nuclear Power Plant in Ukraine, which was still a part of the former Soviet Union. While operating at low power during an unauthorized experiment with some of its safety devices shut off, one of the reactors at the plant became unstable. Its chain reaction became uncontrollable and increased to a level far beyond what the reactor was designed for. The steam pressure in the reactor rose to between 100 and 500 times the full power pressure and ruptured the reactor. Because the reactor was not enclosed in a containment building, a large amount of radioactive material spewed out, and additional fission products were released, as the graphite (carbon) moderator of the core ignited and burned. The fire was controlled, but over 200 plant workers and firefighters developed acute radiation sickness and at least 32 soon died from the effects of the radiation. It is predicted that about 4000 more deaths will occur among emergency workers and former Chernobyl residents from radiation-induced cancer and leukemia. The reactor has since been encapsulated in steel and concrete, a now-decaying structure known as the sarcophagus. Almost 30 years later, significant radiation problems still persist in the area, and Chernobyl largely remains a wasteland.
In 2011, the Fukushima Daiichi Nuclear Power Plant in Japan was badly damaged by a 9.0-magnitude earthquake and resulting tsunami. Three reactors up and running at the time were shut down automatically, and emergency generators came online to power electronics and coolant systems. However, the tsunami quickly flooded the emergency generators and cut power to the pumps that circulated coolant water through the reactors. High-temperature steam in the reactors reacted with zirconium alloy to produce hydrogen gas. The gas escaped into the containment building, and the mixture of hydrogen and air exploded. Radioactive material was released from the containment vessels as the result of deliberate venting to reduce the hydrogen pressure, deliberate discharge of coolant water into the sea, and accidental or uncontrolled events.
An evacuation zone around the damaged plant extended over 12.4 miles away, and an estimated 200,000 people were evacuated from the area. All 48 of Japan’s nuclear power plants were subsequently shut down, remaining shuttered as of December 2014. Since the disaster, public opinion has shifted from largely favoring to largely opposing increasing the use of nuclear power plants, and a restart of Japan’s atomic energy program is still stalled ( Figure 21.22 ).
The energy produced by a reactor fueled with enriched uranium results from the fission of uranium as well as from the fission of plutonium produced as the reactor operates. As discussed previously, the plutonium forms from the combination of neutrons and the uranium in the fuel. In any nuclear reactor, only about 0.1% of the mass of the fuel is converted into energy. The other 99.9% remains in the fuel rods as fission products and unused fuel. All of the fission products absorb neutrons, and after a period of several months to a few years, depending on the reactor, the fission products must be removed by changing the fuel rods. Otherwise, the concentration of these fission products would increase and absorb more neutrons until the reactor could no longer operate.
Spent fuel rods contain a variety of products, consisting of unstable nuclei ranging in atomic number from 25 to 60, some transuranium elements, including plutonium and americium, and unreacted uranium isotopes. The unstable nuclei and the transuranium isotopes give the spent fuel a dangerously high level of radioactivity. The long-lived isotopes require thousands of years to decay to a safe level. The ultimate fate of the nuclear reactor as a significant source of energy in the United States probably rests on whether or not a politically and scientifically satisfactory technique for processing and storing the components of spent fuel rods can be developed.
Explore the information in this link to learn about the approaches to nuclear waste management.
Nuclear Fusion and Fusion Reactors
The process of converting very light nuclei into heavier nuclei is also accompanied by the conversion of mass into large amounts of energy, a process called fusion . The principal source of energy in the sun is a net fusion reaction in which four hydrogen nuclei fuse and produce one helium nucleus and two positrons. This is a net reaction of a more complicated series of events:
A helium nucleus has a mass that is 0.7% less than that of four hydrogen nuclei; this lost mass is converted into energy during the fusion. This reaction produces about 3.6 × × 10 11 kJ of energy per mole of 2 4 He 2 4 He produced. This is somewhat larger than the energy produced by the nuclear fission of one mole of U-235 (1.8 × × 10 10 kJ), and over 3 million times larger than the energy produced by the (chemical) combustion of one mole of octane (5471 kJ).
It has been determined that the nuclei of the heavy isotopes of hydrogen, a deuteron, 1 2 H 1 2 H and a triton, 1 3 H , 1 3 H , undergo fusion at extremely high temperatures (thermonuclear fusion). They form a helium nucleus and a neutron:
This change proceeds with a mass loss of 0.0188 amu, corresponding to the release of 1.69 × × 10 9 kilojoules per mole of 2 4 He 2 4 He formed. The very high temperature is necessary to give the nuclei enough kinetic energy to overcome the very strong repulsive forces resulting from the positive charges on their nuclei so they can collide.
Useful fusion reactions require very high temperatures for their initiation—about 15,000,000 K or more. At these temperatures, all molecules dissociate into atoms, and the atoms ionize, forming plasma. These conditions occur in an extremely large number of locations throughout the universe—stars are powered by fusion. Humans have already figured out how to create temperatures high enough to achieve fusion on a large scale in thermonuclear weapons. A thermonuclear weapon such as a hydrogen bomb contains a nuclear fission bomb that, when exploded, gives off enough energy to produce the extremely high temperatures necessary for fusion to occur.
Another much more beneficial way to create fusion reactions is in a fusion reactor , a nuclear reactor in which fusion reactions of light nuclei are controlled. Because no solid materials are stable at such high temperatures, mechanical devices cannot contain the plasma in which fusion reactions occur. Two techniques to contain plasma at the density and temperature necessary for a fusion reaction are currently the focus of intensive research efforts: containment by a magnetic field and by the use of focused laser beams ( Figure 21.23 ). A number of large projects are working to attain one of the biggest goals in science: getting hydrogen fuel to ignite and produce more energy than the amount supplied to achieve the extremely high temperatures and pressures that are required for fusion. At the time of this writing, there are no self-sustaining fusion reactors operating in the world, although small-scale controlled fusion reactions have been run for very brief periods.
As an Amazon Associate we earn from qualifying purchases.
This book may not be used in the training of large language models or otherwise be ingested into large language models or generative AI offerings without OpenStax's permission.
Want to cite, share, or modify this book? This book uses the Creative Commons Attribution License and you must attribute OpenStax.
Access for free at https://openstax.org/books/chemistry-2e/pages/1-introduction
- Authors: Paul Flowers, Klaus Theopold, Richard Langley, William R. Robinson, PhD
- Publisher/website: OpenStax
- Book title: Chemistry 2e
- Publication date: Feb 14, 2019
- Location: Houston, Texas
- Book URL: https://openstax.org/books/chemistry-2e/pages/1-introduction
- Section URL: https://openstax.org/books/chemistry-2e/pages/21-4-transmutation-and-nuclear-energy
© Jan 8, 2024 OpenStax. Textbook content produced by OpenStax is licensed under a Creative Commons Attribution License . The OpenStax name, OpenStax logo, OpenStax book covers, OpenStax CNX name, and OpenStax CNX logo are not subject to the Creative Commons license and may not be reproduced without the prior and express written consent of Rice University.
Browse Course Material
Course info.
- Prof. Michael Short
Departments
- Nuclear Science and Engineering
As Taught In
- Nuclear Engineering
- Nuclear Physics
Learning Resource Types
Introduction to nuclear engineering and ionizing radiation, lecture 21: neutron transport.
Description: The full, seven-dimensional neutron transport equation is developed from physical intuition, and putting that intuition into math. Aspects of neutron creation and transport are introduced as needed—neutron energy birth spectrum, flux, current, and many different types of neutron cross sections (fission, capture, scattering, total). It all comes down to balance—we equate the ways in which a control volume (like a reactor) can gain and lose neutrons of a certain energy, in a certain direction.
Instructor: Michael Short
Note: To report potential content errors, please use this form .
- Download video
- Download transcript

You are leaving MIT OpenCourseWare
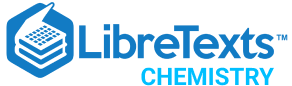
- school Campus Bookshelves
- menu_book Bookshelves
- perm_media Learning Objects
- login Login
- how_to_reg Request Instructor Account
- hub Instructor Commons
- Download Page (PDF)
- Download Full Book (PDF)
- Periodic Table
- Physics Constants
- Scientific Calculator
- Reference & Cite
- Tools expand_more
- Readability
selected template will load here
This action is not available.
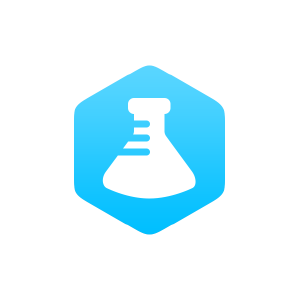
10.7: Energy from the Nucleus
- Last updated
- Save as PDF
- Page ID 475612
Learning Objectives
- Explain where nuclear energy comes from.
- Describe the difference between fission and fusion.
- Know key examples of nuclear fission and nuclear fusion.
A combination of radiochemistry and radiation chemistry is used to study nuclear reactions such as fission and fusion. Some early evidence for nuclear fission was the formation of a short-lived radioisotope of barium which was isolated from neutron irradiated uranium ( 139 Ba, with a half-life of 83 minutes and 140 Ba, with a half-life of 12.8 days, are major fission products of uranium). Nuclear fission is the splitting of an atomic nucleus. In nuclear weapons and reactors, neutrons hit unstable nuclei to form smaller atoms. Nuclear Fusion is the bringing together of two atomic nuclei to form a larger atom. Figure \(\PageIndex{1}\) illustrates the difference between nuclear fission and nuclear fusion.
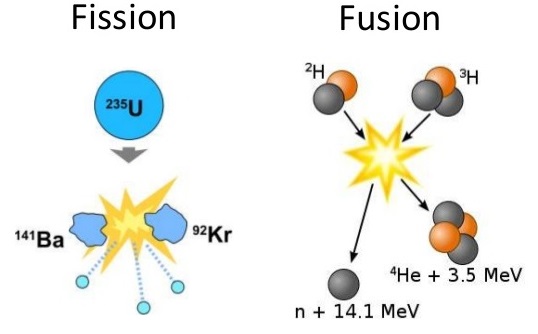
Einstein and the Equivalence of Mass and Energy
Nuclear changes occur with a simultaneous release of energy. Where does this energy come from? If we could precisely measure the masses of the reactants and products of a nuclear reaction, we would notice that the amount of mass drops slightly in the conversion from reactants to products. Consider the following nuclear equation, in which the molar mass of each species is indicated to four decimal places:
\[_{235.0439}^{235}\textrm{U}\rightarrow _{138.9088}^{139}\textrm{Ba}+_{93.9343}^{94}\textrm{Kr}+_{2\times 1.0087}^{2^{1}}\textrm{n} \nonumber \]
If we compare the mass of the reactant (235.0439) to the masses of the products (sum=234.8605) we notice a mass difference of -0.1834 g or -0.0001834 kg. Where did this mass go?
According to Albert Einstein's theory of relativity, energy ( E ) and mass ( m ) are related by the following equation:
\[E = mc^2 \nonumber \]
where c is the speed of light, or \[c=3.00\times 10^{8}\, m/s \nonumber \]
In the course of the chemical reaction for uranium, the mass difference is converted to energy, which is given off by the reaction:
\[E=(-0.0001834\, kg)(3.00\times 10^{8}\, m/s)^{2}=-1.65\times 10^{13}J=-1.65\times 10^{10}kJ \nonumber \]
(For the units to work out, mass must be expressed in units of kilograms.) That is, 16.5 billion kJ of energy is given off every time 1 mol of uranium-235 undergoes this nuclear reaction. This is an extraordinary amount of energy. Compare it to combustion reactions of hydrocarbons, which give off about 650kJ/mol of energy for every CH 2 unit in the hydrocarbon-on the order of hundreds of kilojoules per mole. Nuclear reactions give off billions of kilojoules per mole.
If this energy could be properly harvested, it would be a significant source of energy for our society. Nuclear energy involves the controlled harvesting of energy from fission reactions. The reaction can be controlled because the fission of uranium-235 (and a few other isotopes, such as plutonium-239) can be artificially initiated by injecting a neutron into a uranium nucleus. The overall nuclear equation, with energy included as a product, is then as follows:
\[_{}^{235}\textrm{U}\: +\: _{ }^{1}\textrm{n}\rightarrow \: _{ }^{139}\textrm{Ba}\: +\: _{ }^{94}\textrm{Kr}\: +\: 3_{ }^{1}\textrm{n} \nonumber \]
Thus by the careful addition of extra neutrons into a sample of uranium, we can control the fission process and obtain energy that can be used for other purposes. (Artificial or induced radioactivity, in which neutrons are injected into a sample of matter that subsequently cause fission, was first demonstrated in 1934 by Irène Joliot-Curie and Frédéric Joliot, the daughter and son-in-law of Marie Curie.)
Binding Energy
The forces that bind nucleons together in an atomic nucleus are much greater than those that bind an electron to an atom through electrostatic attraction. This is evident by the relative sizes of the atomic nucleus and the atom (\(10^{-15}\) and \(10^{-10}\)m, respectively). The energy required to pry a nucleon from the nucleus is therefore much larger than that required to remove (or ionize) an electron in an atom. In general, all nuclear changes involve large amounts of energy per particle undergoing the reaction. This has numerous practical applications.
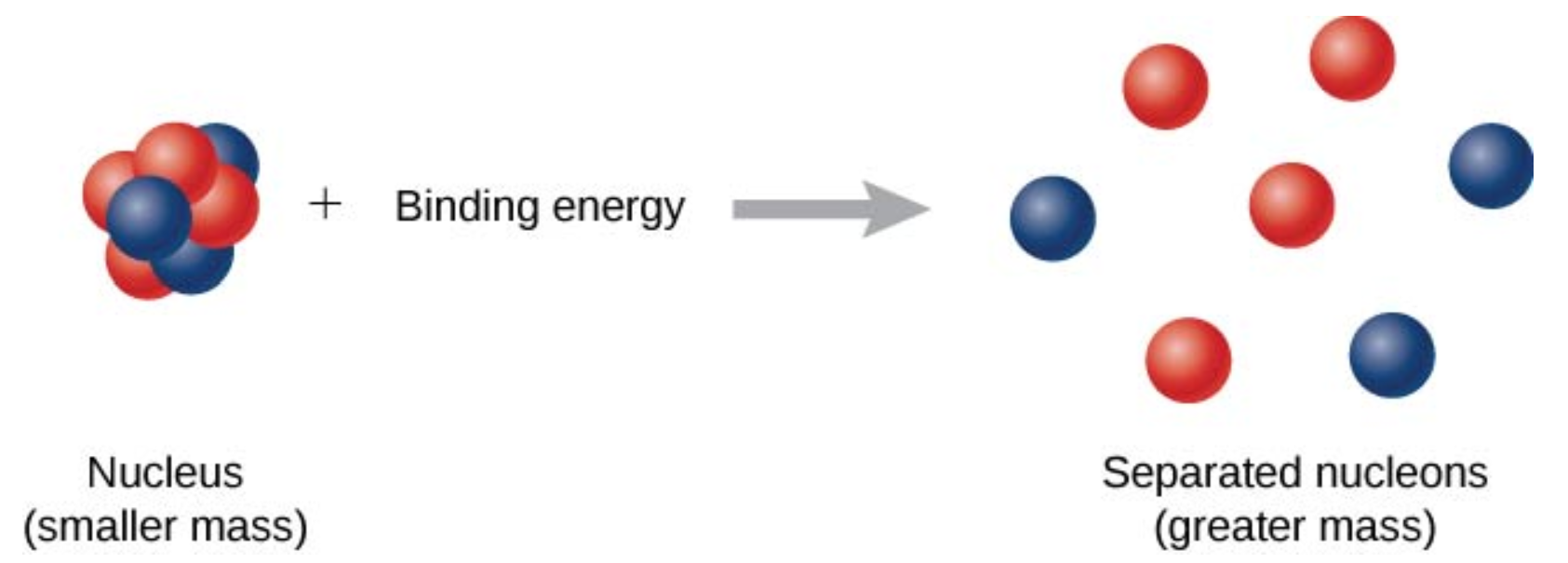
As shown in figure \(\PageIndex{2}\), energy is put into the system to break apart the nucleus. The amount of energy required is called the total binding energy (BE), \(E_b\). The binding energy is equal to the amount of energy released in forming the nucleus, and is therefore given by
\[E_b = (\Delta m)c^2. \label{BE} \]
In nuclear physics, one of the most important experimental quantities is the binding energy per nucleon (BEN) , which is defined by
\[BEN = \dfrac{E_b}{A} \label{BEN} \]
This quantity is the average energy required to remove an individual nucleon (proton or neutron) from a nucleus—analogous to the ionization energy of an electron in an atom. If the BEN is relatively large, the nucleus is relatively stable. BEN values are estimated from nuclear scattering experiments.
Figure \(\PageIndex{3}\) shows the relative binding energies for various isotopes. Of these elements, fission requires heavy, unstable nuclei. This means selected atoms would have low binding energies and would have large atomic masses. Nuclei that are larger than Fe-56 may undergo fission. Uranium-238 and uranium-235 both have lower binding energies with heavy masses-.
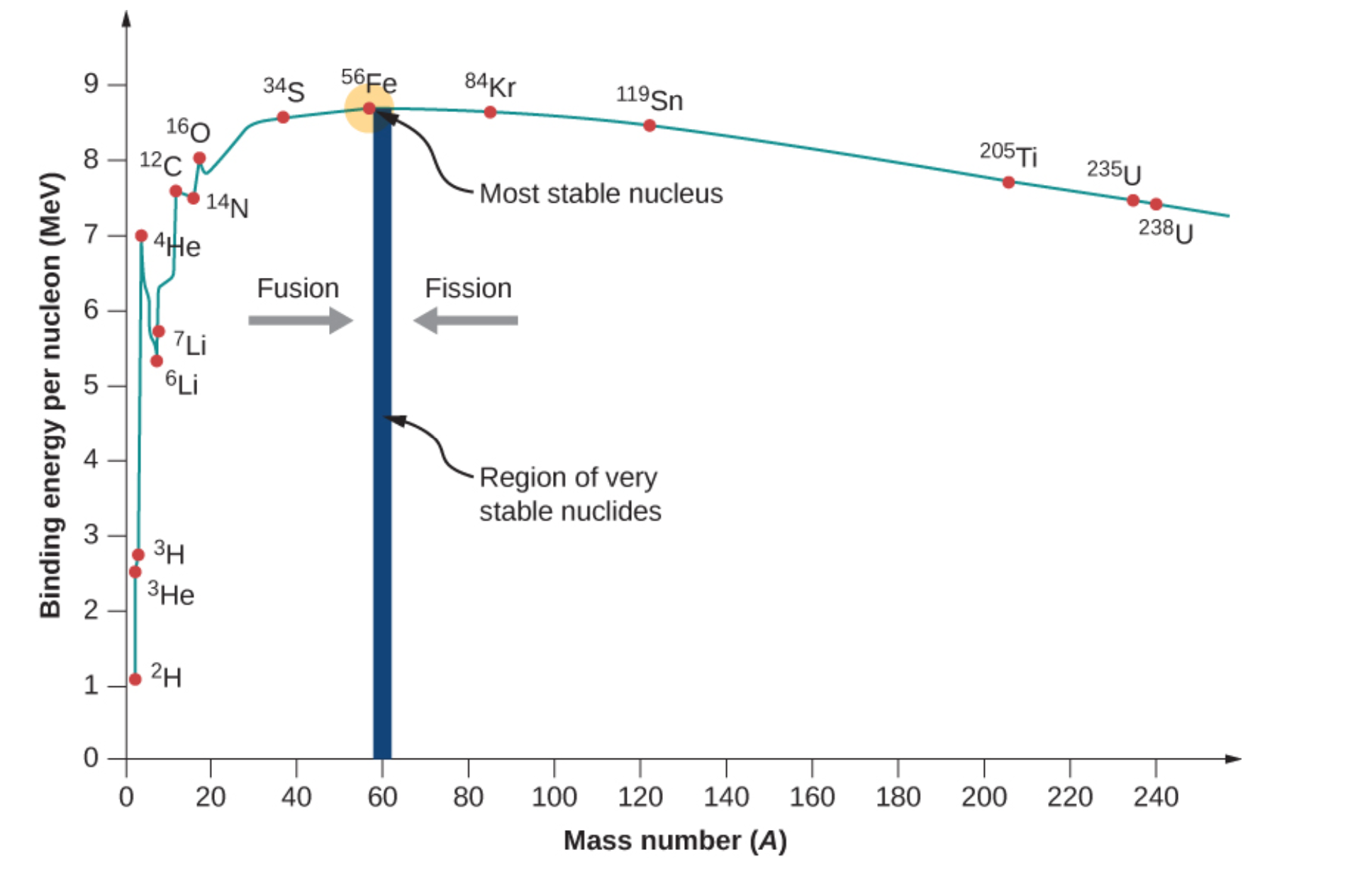
These two isotopes would be suitable for splitting based on these requirements. Of these two isotopes, only uranium-235 is readily fissionable. This is due to odd neutron count contributing to additional instability. Another fissionable isotope not shown in Figure \(\PageIndex{3}\) is plutonium-239. This is a synthetic isotope produced by transmutation and decay reactions. Like uranium-235, it has low binding energy, high mass, and an odd number of neutrons. U-235 and Pu-239 are used in atomic bombs (fission based) and nuclear reactors.
Positively charged centers of atoms make nuclear fusion extremely difficult. For this reason, smaller atoms are suitable for fusion reactions. In addition, these particular isotopes need to have low binding energies in order to undergo fusion. Atoms that possess these two qualities are H-2 and H-3. For fusion to occur, extreme temperatures are required to fuse these deuterium and tritium together.
As we will see, the BEN-versus- A graph implies that nuclei divided or combined release an enormous amount of energy. This is the basis for a wide range of phenomena, from the production of electricity at a nuclear power plant to sunlight.
Nuclear Fission
In both fission and fusion, large amounts of energy are given off in the form of heat, light, and gamma radiation. Italian physicist, Enrico Fermi, performed the first fission reaction in 1934. He was unaware that he had split a uranium atom into two smaller nuclei. Nuclear fission of heavy elements was discovered on December 17, 1938 by German Otto Hahn and his assistant Fritz Strassmann, and explained theoretically in January 1939 by Lise Meitner (Figure \(\PageIndex{4}\)) and her nephew Otto Robert Frisch. Frisch named the process "fission" by analogy with biological fission of living cells.
Image take from: https://upload.wikimedia.org/wikiped...ner12crop2.J
Since then, fission has been observed in many other isotopes, including most actinide isotopes that have an odd number of neutrons. A typical nuclear fission reaction is shown in Figure \(\PageIndex{5}\).
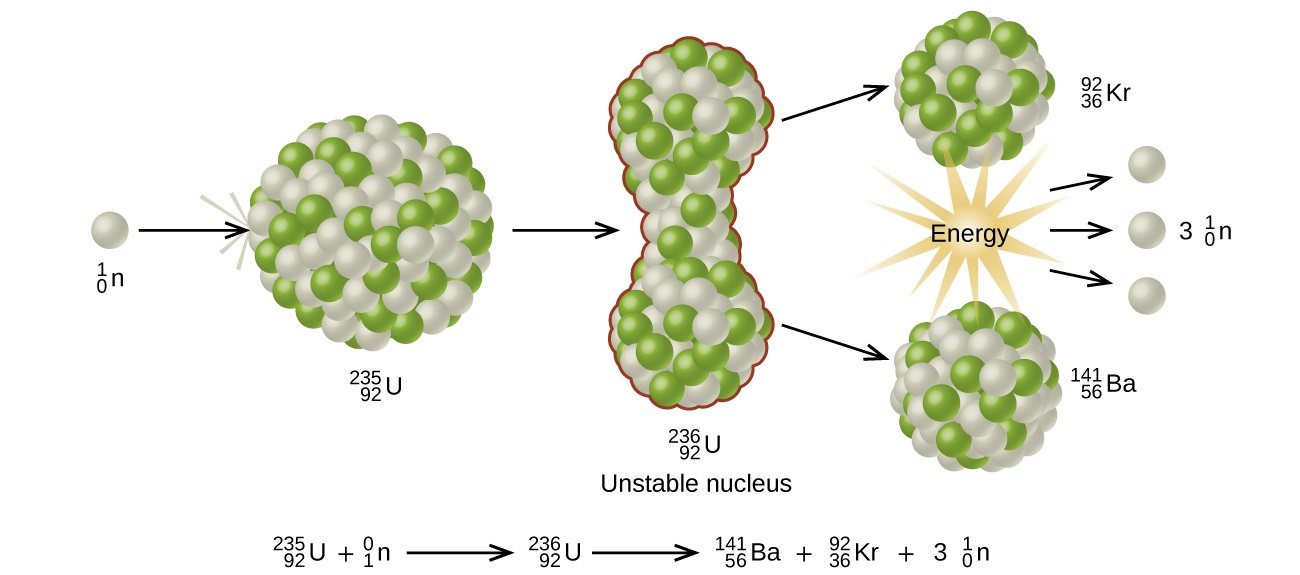
Among the products of Meitner, Hahn, and Strassman’s fission reaction were barium, krypton, lanthanum, and cerium, all of which have nuclei that are more stable than uranium-235. Since then, hundreds of different isotopes have been observed among the products of fissionable substances.
Fission has been used in nuclear weapons and powers all nuclear reactors. Approximately fifty-five countries worldwide possess fission technology in the form of research or energy reactors. Less than ten of these countries have fission weapons.
A tremendous amount of energy is produced by the fission of heavy elements. For instance, when one mole of U-235 undergoes fission, the products weigh about 0.2 grams less than the reactants; this “lost” mass is converted into a very large amount of energy, about 1.8 × 10 10 kJ per mole of U-235. Nuclear fission reactions produce incredibly large amounts of energy compared to chemical reactions. The fission of 1 kilogram of uranium-235, for example, produces about 2.5 million times as much energy as is produced by burning 1 kilogram of coal.

Nuclear Chain Reaction
As described earlier, when undergoing fission U-235 produces two “medium-sized” nuclei, and two or three neutrons. These neutrons may then cause the fission of other uranium-235 atoms, which in turn provide more neutrons that can cause fission of even more nuclei, and so on. If this occurs, we have a nuclear chain reaction (Figure \(\PageIndex{6}\)). On the other hand, if too many neutrons escape the bulk material without interacting with a nucleus, then no chain reaction will occur.

Material that can sustain a nuclear fission chain reaction is said to be fissile or fissionable . (Technically, fissile material can undergo fission with neutrons of any energy, whereas fissionable material requires high-energy neutrons.) Nuclear fission becomes self-sustaining when the number of neutrons produced by fission equals or exceeds the number of neutrons absorbed by splitting nuclei plus the number that escape into the surroundings. The amount of a fissionable material that will support a self-sustaining chain reaction is a critical mass . An amount of fissionable material that cannot sustain a chain reaction is a subcritical mass . An amount of material in which there is an increasing rate of fission is known as a supercritical mass . The critical mass depends on the type of material: its purity, the temperature, the shape of the sample, and how the neutron reactions are controlled (Figure \(\PageIndex{7}\)).
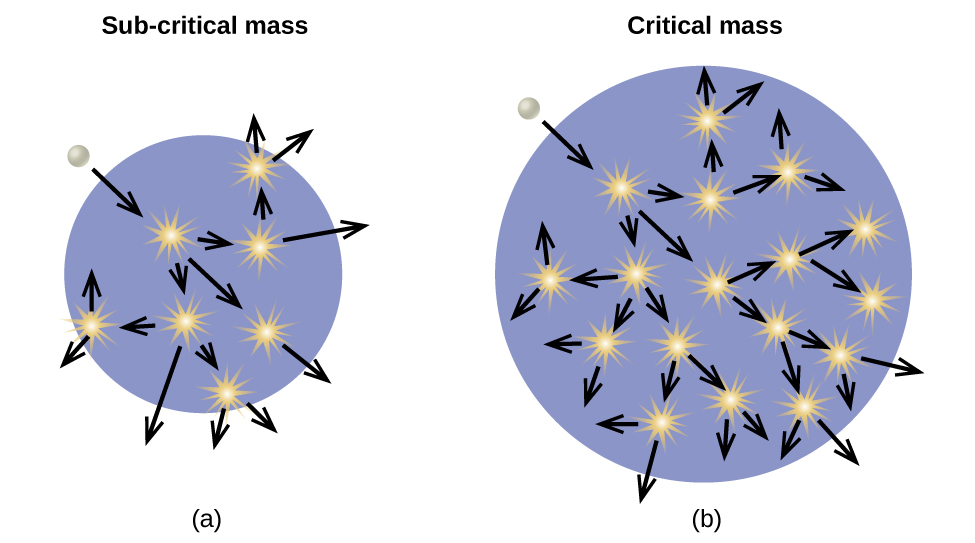
Thermonuclear Reactions
Thermonuclear fusion is a way to achieve nuclear fusion by using extremely high temperatures. There are two forms of thermonuclear fusion: uncontrolled , in which the resulting energy is released in an uncontrolled manner, as it is in thermonuclear weapons ("hydrogen bombs") and in most stars; and controlled , where the fusion reactions take place in an environment allowing some or all of the energy released to be harnessed for constructive purposes. The discussion below is limited to the fusion process that powers the sun and the stars.
The prime energy producer in the sun is the fusion of hydrogen to form helium, which occurs at a solar-core temperature of 14 million kelvin. The net result is the fusion of four protons into one alpha particle, with the release of two positrons, two neutrinos (which changes two of the protons into neutrons), and energy (Figure \(\PageIndex{8}\)).
This is a net reaction of a more complicated series of events:
\[\ce{4^1_1H ⟶ ^4_2He + 2 ^1_{0}n} \nonumber \]
A helium nucleus has a mass that is 0.7% less than that of four hydrogen nuclei; this lost mass is converted into energy during the fusion. This reaction produces about 3.6 × 10 11 kJ of energy per mole of \(\ce{^4_2He}\) produced. This is somewhat larger than the energy produced by the nuclear fission of one mole of U-235 (1.8 × 10 10 kJ), and over 3 million times larger than the energy produced by the (chemical) combustion of one mole of octane (5471 kJ).
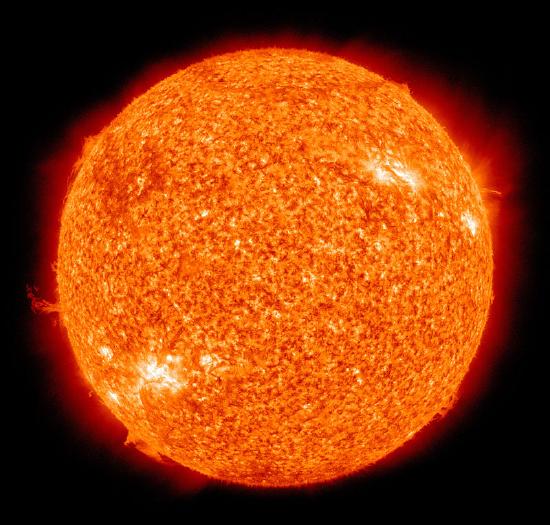
- Nuclear energy comes from tiny mass changes in nuclei as radioactive processes occur.
- In fission, large nuclei break apart and release energy; in fusion, small nuclei merge together and release energy.
- The continues process whereby neutrons produced from the initial fission of a large nucleus like U-235 cause further fission of another nucleus.
- In a critical mass, a large enough number of neutrons in the fissile material induce fission to create a chain reaction.
- The most important fusion process in nature is the one that powers the stars. The net result is the fusion of four protons into one alpha particle, with the release of two positrons, two neutrinos (which changes two of the protons into neutrons), and energy
Contributors and Attributions
Elizabeth R. Gordon (Furman University)
Paul Flowers (University of North Carolina - Pembroke), Klaus Theopold (University of Delaware) and Richard Langley (Stephen F. Austin State University) with contributing authors. Textbook content produced by OpenStax College is licensed under a Creative Commons Attribution License 4.0 license. Download for free at http://cnx.org/contents/[email protected] ).
- TextMap: Beginning Chemistry (Ball et al.)
Marisa Alviar-Agnew ( Sacramento City College )
Generation IV Nuclear Reactors
- An international task force is sharing R&D to develop six generation IV nuclear reactor technologies. Four are fast neutron reactors.
- All of these operate at higher temperatures than today's reactors. In particular, four are designated for hydrogen production.
- All six systems represent advances in sustainability, economics, safety, reliability and proliferation-resistance.
- Europe is pushing ahead with three of the fast reactor designs.
- A separate programme set up by regulators aims to develop multinational regulatory standards for Generation IV reactors.
Generation IV International Forum
The Generation IV International Forum (GIF) was initiated by the US Department of Energy in 2000 and formally chartered in mid-2001. It is an international collective representing governments of 13 countries where nuclear energy is significant now and also seen as vital for the future. Most are committed to joint development of the next generation of nuclear technology. The original charter members of GIF are Argentina, Brazil, Canada, France, Japan, South Korea, South Africa, the UK and the USA. They have been joined by Switzerland, China, Russia, Australia and, through the Euratom research and training programme, the European Union. The purpose of GIF is to share R&D rather than build reactors.
Most of the countries are party to the 2005 Framework Agreement, which formally commits them to participate in the development of one or more Generation IV systems selected by GIF for further R&D. Argentina, Australia and Brazil did not sign the Framework Agreement, and the UK withdrew from it. Russia formalized its accession to the Framework Agreement in August 2009 as its tenth member, with Rosatom as implementing agent. In 2011 the 13 members decided to modify and extend the GIF charter indefinitely. (Australia joined as the 13th GIF member country in June 2016.) In February 2015 the Framework Agreement was extended for ten years, with Rosatom signing for the extension in June, and Euratom in November 2016. In November 2018, the UK became the 12th member to ratify the Framework Agreement. Argentina and Brazil remain non-active members.
In May 2019 Terrestrial Energy, the Canadian developer of a molten salt reactor, became the first private sector company to join GIF.
Earlier in 2005 the technical secretariat transferred to the OECD Nuclear Energy Agency (NEA), alongside that of MDEP (see section below ), and from mid-2015 also the International Framework for Nuclear Energy Cooperation (IFNEC). The NEA in Paris provides the technical secretariat to support the system steering committees, project management boards, modelling working groups and task forces for GIF.
After some two years' deliberation and review of about one hundred concepts, late in 2002 GIF (then representing ten countries) announced the selection of six reactor technologies which they believe represent the future shape of nuclear energy (see section below ). These were selected on the basis of being clean, safe and cost-effective means of meeting increased energy demands on a sustainable basis, while being resistant to diversion of materials for weapons proliferation and secure from terrorist attacks. They are the subject of further development internationally, with expenditure of about $6 billion over 15 years. About 80% of the cost is being met by the USA, Japan and France.
In February 2005 five of the participants signed an agreement to take forward the R&D on the six technologies. The USA, Canada, France, Japan and UK agreed to undertake joint research and exchange technical information.
In addition to selecting these six concepts for deployment between 2010 and 2030, GIF recognised a number of International Near-Term Deployment advanced reactors available before 2015. See also information page on Advanced Nuclear Power Reactors .
Most of the six systems employ a closed fuel cycle to maximise the resource base and minimise high-level wastes to be sent to a repository. Three of the six are fast neutron reactors and one can be built as a fast reactor, one is described as epithermal, and only two operate with slow neutrons like today's plants. See also information page on Fast Neutron Reactors .
Only one is cooled by light water, two are helium-cooled and the others have lead-bismuth, sodium or fluoride salt coolant. The latter three operate at low pressure, with significant safety advantage. The last has the uranium fuel dissolved in the circulating coolant. Temperatures range from 510°C to 1000°C, compared with less than 330°C for today's light water reactors, and this means that four of them can be used for thermochemical hydrogen production. See also information page on Hydrogen Production and Uses .
The sizes range from 150 to 1500 MWe (or equivalent thermal), with the lead-cooled one optionally available as a 50-150 MWe 'battery' with long core life (15-20 years without refuelling) as replaceable cassette or entire reactor module. This is designed for distributed generation or desalination.
At least four of the systems have significant operating experience already in most respects of their design, which provides a good basis for further R&D and is likely to mean that they can be in commercial operation before 2030.
However, it is significant that to address non-proliferation concerns, the fast neutron reactors are not conventional fast breeders ( i.e. they do not have a blanket assembly where plutonium-239 is produced). Instead, plutonium production takes place in the core, where burn-up is high and the proportion of plutonium isotopes other than Pu-239 remains high. In addition, new electrometallurgical reprocessing technologies will enable the fuel to be recycled without separating the plutonium.
Closely related to GIF, but more focused on Generation III immediately, is the Multinational Design Evaluation Programme ( MDEP ) set up by the regulators. It was launched in 2006 by the US Nuclear Regulatory Commission (NRC) and the French Nuclear Safety Authority (ASN) to develop innovative approaches to leverage the resources and knowledge of national regulatory authorities reviewing new reactor designs. It involves the IAEA and 15 countries, and its secretariat is with the OECD Nuclear Energy Agency. Ultimately it aims to develop multinational regulatory standards for design of Generation IV reactors. The US NRC has proposed a three-stage process culminating in international design certification for new reactor types, notably Generation IV types.
The MDEP pools the resources of its member nuclear regulatory authorities for the purpose of i) cooperating on safety reviews of designs of nuclear reactors that are under construction and undergoing licensing in several countries, and ii) exploring opportunities and potential for harmonization of regulatory requirements and practices. It also produces reports and guidance documents that are shared internationally beyond the MDEP membership. It has five design-specific working groups: EPR, AP1000, APR1400, VVER, ABWR, and one issue-specific one: vendor inspection cooperation. It earlier had working groups for digitial I&C, and mechanical codes and standards
In relation to Generation IV reactors, the NRC has called for countries involved in their development to develop common design requirements so that regulatory standards can be harmonized. The NRC has published its draft design requirements.
Meanwhile the MDEP is being used to share information among countries engaged in certifying particular new reactor designs, notably the EPR, AP1000, APR1400, VVER designs and ABWR-ESBWR. MDEP members are looking at different design codes to ensure that consistently high safety standards are achieved in different countries. Harmonization of design requirements will ultimately assist this, in the same way as has been achieved in civil aviation.
Associated ongoing programmes
While Russia was not initially part of GIF, one design corresponds with the BREST reactor being developed there, and Russia is now the main operator of the sodium-cooled fast reactor for electricity – another of the technologies put forward by GIF.
India is also not involved with GIF but is developing its own advanced technology to utilise thorium as a nuclear fuel. A three-stage program has the first stage well-established, with pressurised heavy water reactors (PHWR) fuelled by natural uranium to generate plutonium. Then fast breeder reactors (FBRs) use this plutonium-based fuel to breed U-233 from thorium, and finally advanced nuclear power systems will use the U-233. The spent fuel will be reprocessed to recover fissile materials for recycling. The two major options for the third stage, while continuing with the PHWR and FBR programmes, are an Advanced Heavy Water Reactor and subcritical Accelerator-Driven Systems.
A major project relevant to several Generation IV designs is investigating the use of actinide-laden fuel assemblies in fast reactors as part of the sodium-cooled fast reactor program. The Global Actinide Cycle International Demonstration (GACID) is being undertaken by France's atomic energy commission (CEA), Japan's Atomic Energy Agency (JAEA) and the US Department of Energy (DOE) under the US Advanced Fuel Cycle Initiative (AFCI). The first stage will lead to demonstration fuel containing minor actinides being used in Japan's Monju reactor.
GIF reactor technologies
There were originally six technologies chosen, but development on one has gone in two directions, so seven are listed in the Table below.
Gas-cooled fast reactor (GFR) . Like other helium-cooled reactors which have operated or are under development, GFRs will be high-temperature units – typically 800-850°C. They employ similar reactor technology to the VHTR, suitable for power generation, thermochemical hydrogen production or other process heat. The reference GFR unit is 2400 MWt/1200 MWe with a core outlet temperature of 850°C, large enough for breakeven breeding, with thick steel reactor pressure vessel and three 800 MWt loops. The high core outlet temperature places significant demands on the fuel to operate continuously with the high power density required for good neutron economy in the core – this is recognised as the greatest challenge in the development of the GFR system. For electricity, an indirect cycle with helium will be on the primary circuit, in the secondary circuit the helium gas will directly drive a gas turbine (Brayton cycle), and a steam cycle will comprise the tertiary circuit. It would have a self-generating (breeding) core with fast neutron spectrum and no fertile blanket. Robust nitride or carbide fuels would include depleted uranium and any other fissile or fertile materials as ceramic pins or plates, with plutonium content of 15 to 20%. As with the SFR, used fuel would be reprocessed onsite and all the actinides recycled repeatedly to minimise production of long-lived radioactive waste.
While General Atomics worked on the design in the 1970s (but not as fast reactor), none has so far been built. It is the only Generation IV design with no operating antecedent. However, a 75 MWt experimental technology demonstration GFR, Allegro, is planned by Euratom. It will incorporate all the architecture and the main materials and components foreseen for the GFR without the power conversion system. The Allegro project has been taken over by the V4G4 partnership in Eastern Europe, with French CEA support and the prepatory phase now extending to 2026. Euratom, France, Japan and Switzerland have signed on to system arrangements for the GFR under the Framework Agreement. See also European programme section below.
An alternative GFR design has lower temperature (600-650ºC) helium cooling in a primary circuit and supercritical CO 2 at 550ºC and 20 MPa in a secondary system for power generation. This reduces the metallurgical and fuel challenges associated with very high temperatures.
The General Atomics (GA) Energy Multiplier Module (EM 2 ) design is a 500 MWt, 240 MWe helium-cooled fast-neutron HTR operating at 850°C and fuelled with used PWR fuel or depleted uranium, plus some low-enriched uranium as starter. GA has teamed up with Chicago Bridge & Iron, Mitsubishi Heavy Industries, and Idaho National Laboratory to develop the EM 2 , but it is not part of the Generation IV programme or mentioned in the 2014 roadmap. Then in October 2020 General Atomics teamed up with Framatome to launch the 50 MWe Fast Modular Reactor (FMR) partly based on EM 2 .
The main research needs, according to the China Academy of Sciences, are fuels, materials and thermal-hydraulics.
Lead-cooled fast reactor (LFR) . The LFR is a flexible fast neutron reactor which can use depleted uranium or thorium fuel matrices, and burn actinides from LWR fuel. Liquid metal (Pb or Pb-Bi eutectic) cooling is at atmospheric pressure by natural convection (at least for decay heat removal). Fuel is metal or nitride, with full actinide recycle from regional or central reprocessing plants. A wide range of unit sizes is envisaged, from factory-built "battery" with 15-20 year life for small grids or developing countries, to modular 300-400 MWe units and large single plants of 1400 MWe. Operating temperature of 550°C is readily achievable but 800°C is envisaged with advanced materials to provide lead corrosion resistance at high temperatures which would enable thermochemical hydrogen production. A two-stage development programme leading to industrial deployment is envisaged: by 2025 for reactors operating with relatively low temperature and power density, and by 2040 for more advanced higher-temperature designs.
This corresponds with Russia's BREST fast reactor technology which is lead-cooled and builds on 80 reactor-years' experience of lead or lead-bismuth cooling, mostly in submarine reactors. However, these propulsion reactors were small, operated at low capacity factors, featured an epithermal (not fast) neutron spectrum and operated at significantly lower temperatures than those anticipated in Generation IV LFRs. More immediately the GIF proposal appeared to arise from two experimental designs: the US STAR and Japan's LSPR, these being lead and lead-bismuth cooled respectively.
Initial development work on the LFR was focused on two pool-type reactors: SSTAR – Small Secure Transportable Autonomous Reactor of 20 MWe in USA; and the European Lead-cooled SYstem (ELSY) or European Lead Fast Reactor (ELFR) of 600 MWe in Europe.* In 2014 the leading developments were Russia’s SVBR-100 and BREST-300, Europe’s 300 MWt ALFRED, and Belgium’s MYRRHA, with R&D focus on fuels and materials corrosion. Russia's SVBR-100 has since been cancelled. In October 2015 Westinghouse announced that it had submitted an LFR project proposal for the DOE’s upcoming investment in advanced reactor concepts demonstrable in the 2035 timeframe.
* SSTAR runs at 564°C and has integral steam generator inside the sealed unit, which would be installed below ground level. After 20 years of operation without refuelling, the whole reactor unit would then be returned for recycling the fuel. The core is one metre high and 1.2 m diameter (20 MWe version). The ELSY or ELFR project is led by Ansaldo Nucleare from Italy and was being financed by Euratom. The 600 MWe design was nearly complete in 2008 and a small-scale demonstration facility was planned, but little has been heard since then. It appears to be superseded by the ALFRED reactor. It runs on MOX fuel (with or without actinides) at 480°C and the molten lead is pumped to eight steam generators. Decay heat removal is by convection.
For the LFR, no system arrangements have been signed, and collaborative R&D is pursued by interested members under the auspices of a provisional steering committee led by Japan and Euratom, joined by Russia in 2011. See also European programme section below and Fast Reactor section in the information page on Nuclear Power in Russia .
The main research needs, according to the China Academy of Sciences, are fuels and materials.
Molten salt reactor (MSR) (now two variants): one a fast reactor with fissile material dissolved in the circulation fuel salt; the other with solid particle fuel in graphite and the salt functioning only as coolant.
In what is considered to be a normal MSR, the uranium fuel is dissolved in the fluoride salt coolant which circulates through graphite core channels to achieve some moderation and an epithermal neutron spectrum. The reference plant is up to 1000 MWe. Fission products are removed continuously and the actinides are fully recycled, while plutonium and other actinides can be added along with U-238, without the need for fuel fabrication. Coolant temperature is 700°C at very low pressure, with 800°C envisaged. A secondary coolant system is used for electricity generation, and thermochemical hydrogen production is also feasible.
Compared with solid-fuelled reactors, MSR systems have lower fissile inventories, no radiation damage constraint on fuel burn-up, no requirement to fabricate and handle solid fuel or solid used fuel, and a homogeneous isotopic composition of fuel in the reactor. These and other characteristics may enable MSRs to have unique capabilities and competitive economics for actinide burning and extending fuel resources.
During the 1960s the USA developed the molten salt fast reactor as the primary back-up option for the conventional fast breeder reactor, and a small prototype was operated for about four years. Recent work has focused on lithium and beryllium fluoride (FLiBe) coolant in a fast neutron spectrum (the MSFR) with dissolved thorium and U-233 fuel. MSFRs have large negative temperature and void coefficients. Other attractive features of the MSR fuel cycle concept include: the high-level waste comprising fission products only, hence shorter-lived radioactivity; small inventory of weapons-fissile material (Pu-242 being the dominant Pu isotope); low fuel use (the French self-breeding variant claims 50kg of thorium and 50kg U-238 per billion kWh); and safety due to passive cooling up to any size.
For the MSR, no system arrangements have been signed, but a memorandum of understanding to allow for collaborative R&D by interested members has been signed by Euratom, France, Russia, Switzerland, Canada, USA and Australia. China, Japan and Korea are observers. Terrestrial Energy joined it in 2019 after two years with observer status. There will be a long lead time to prototypes, and the R&D orientation has changed since the project was set up, due to increased interest. It now has two baseline concepts:
- The Molten Salt Fast Neutron Reactor (MSFR), which will take in thorium fuel cycle, recycling of actinides, closed Th/U fuel cycle with no U enrichment, with enhanced safety and minimal wastes.
- The Advanced High-Temperature Reactor (AHTR) – also known as the fluoride salt-cooled high-temperature reactor (FHR) – with the same graphite and solid fuel core structures as the VHTR and molten salt as coolant instead of helium, enabling power densities 4 to 6 times greater than HTRs and power levels up to 4000 MWt with passive safety systems. The TMSR Research Centre is constructing a small solid-fuel simulator (TMSR-SF0) at Shanghai Institute of Nuclear Applied Physics (SINAP, under the China Academy of Sciences) with a 2020 target for operation. It will be followed by a 10 MWt prototype, TMSR-SF1.
The GIF 2014 Roadmap said that a lot of work needed to be done on salts before demonstration reactors were operational, and suggested 2025 as the end of the viability R&D phase.
The main research needs, according to the China Academy of Sciences, which leads world R&D on them, are fuel treatment, materials and reliability.
Sodium-cooled fast reactor (SFR) . The SFR uses liquid sodium as the reactor coolant, allowing high power density with low coolant volume, at low pressure. It builds on some 390 reactor-years experienced with sodium-cooled fast neutron reactors over five decades and in eight countries, and was initially the main technology of interest in GIF, and remains at the forefront despite needing a sealed coolant system. A variety of fuels is possible. Most SFR plants so far have had a core plus blanket configuration, but new designs are likely to have all the neutron action in the core. Other R&D is focused on safety in loss of coolant scenarios, and improved fuel handling.
The SFR utilises depleted uranium as the fuel matrix and has a coolant temperature of 500-550°C enabling electricity generation via a secondary sodium circuit, the primary one being at near atmospheric pressure. Three variants are proposed: a 50-150 MWe modular type with actinides incorporated into a U-Pu metal fuel requiring electrometallurgical processing (pyroprocessing) integrated on site; a 300-1500 MWe pool-type version of this; and a 600-1500 MWe loop-type with conventional MOX fuel, potentially with minor actinides, and advanced aqueous reprocessing in central facilities elsewhere.
Early in 2008, the USA, France and Japan signed an agreement to expand their cooperation on the development of sodium-cooled fast reactor technology. The agreement relates to their collaboration in the International Framework for Nuclear Energy Cooperation (IFNEC), (formerly Global Nuclear Energy Partnership), aimed at closing the nuclear fuel cycle through the use of advanced reprocessing and fast reactor technologies, and seeks to avoid duplication of effort.
One SFR is operational: the BN-800 at Beloyarsk in Russia (grid connection 2015). A second is under construction: Kalpakkam PFBR of 500 MWe in India (delayed, now expected in 2021). The BN-800 is largely an experimental reactor for fast reactor fuels. GIF observes that the technology is "deployable in the very near-term for actinide management." Much of the ongoing R&D focus will be on fuels.
Euratom, China, France, Japan, Korea and the USA have signed on to system arrangements for the SFR under the Framework Agreement, and in 2011 Russia joined them. Several project arrangements (PA) are within the SFR system: the Safety and Operation PA; the Advanced Fuel PA; the Global Actinide Cycle International Demonstration (GACID) PA; the Component Design and Balance-Of-Plant PA; and the System Integration and Assessment PA. See also European programme section below.
The main research needs, according to the China Academy of Sciences, are fuels and advanced recycle options.
Supercritical water-cooled reactor (SCWR) . This is a very high-pressure water-cooled reactor which operates above the thermodynamic critical point of water (374ºC, 22 MPa) to give a thermal efficiency about one-third higher than today's light water reactors from which the design evolves. The supercritical water (25 MPa and 510-550°C) directly drives the turbine, without any secondary steam system,* simplifying the plant. Two design options are considered: pressure vessel and pressure tube. Passive safety features are similar to those of simplified boiling water reactors. Fuel is uranium oxide, enriched in the case of the open fuel cycle option. The core may use thermal neutron spectrum with light or heavy water moderation, or be a fast reactor with full actinide recycle based on conventional reprocessing. Since the SCWR builds both on much BWR experience and that from hundreds of fossil-fired power plants operated with supercritical water, it can readily be developed.
* Today's supercritical coal-fired plants use supercritical water around 25 MPa which have "steam" temperatures of 500 to 600ºC and can give 45% thermal efficiency. At ultra supercritical levels (30+ MPa), 50% thermal efficiency may be attained. Over 400 such plants are operating worldwide. China’s Waigaoqiao 3 (2x1000 MWe) near Shanghai operates at 600°C and 27.6 MPa with 44.5% thermal efficiency.
Supercritical fluids are those above the thermodynamic critical point, defined as the highest temperature and pressure at which gas and liquid phases can co-exist in equilibrium. They have properties between those of gas and liquid. For water the critical point is at 374°C and 22 MPa, giving it a "steam" density one-third that of the liquid so that it can drive a turbine in a similar way to normal steam.
Japanese studies on a pressure vessel design have confirmed a target thermal efficiency of 44% with a core outlet temperature of 500°C, and estimate a potential cost reduction of 30% compared with present PWRs. Safety features are expected to be similar to ABWRs. Canada is developing a pressure tube design with heavy water moderation.
Euratom, Canada and Japan have signed on to system arrangements for the SCWR under the Framework Agreement, in 2011 Russia joined them, followed by China in 2014. Project arrangements are pending for thermal-hydraulics and safety. Pre-conceptual SCWR designs include Candu (Canada), LWR (Euratom) and fast neutron (Japan).
The main research needs, according to the China Academy of Sciences, are materials and thermal-hydraulics.
Very high-temperature gas reactor (VHTR) . These are graphite-moderated, helium-cooled reactors, based on substantial experience.
The core can be built of prismatic blocks such as the Japanese HTTR and General Atomics' earlier GTMHR design and others in Russia, or it may be pebble bed such as the Chinese HTR-10 or HTR-PM and the PBMR formerly under development in South Africa. Outlet temperature of over 900°C and aiming for 1000ºC enables thermochemical hydrogen production via an intermediate heat exchanger, with electricity cogeneration, or direct high-efficiency driving of a gas turbine (Brayton cycle). At lower outlet temperatures, the Rankine steam cycle may be used for electricity generation, and this is the focus for demonstration projects. Modules of 600 MW thermal are envisaged.
There is some flexibility in fuels, but no recycle initially. Fuel is in the form of TRISO (tristructural-isotropic) particles less than a millimetre in diameter. Each has a kernel (ca. 0.5 mm) of uranium oxycarbide (or uranium dioxide), with the uranium enriched up to 20% U-235, though normally less. This is surrounded by layers of carbon and silicon carbide, giving a containment for fission products which is stable to over 1600°C. The particles may be incorporated in billiard ball sized pebbles, or in prismatic graphite blocks. The VHTR has potential for high burn-up (150-200 GWd/t), completely passive safety, low operation and maintenance costs, and modular construction.
The 2014 GIF Roadmap says that a 600 MWt VHTR dedicated to hydrogen production could yield over two million normal cubic metres per day. An R&D priority is qualification of TRISO fuel for operation up to 1250°C and 200 GWd/t burn-up, though US development has attained this, and also its robustness for hundreds of hours at 1,600, 1,700 and 1,800°C. However, in the short term, electricity production and industrial processes based on high temperature steam that require outlet temperatures of (700-850°C) hold most potential.
One question to be resolved regards used fuel. Some synergy with used LWR fuel is possible, and the graphite may be recycled, but much remains to be worked out.
Euratom, France, Japan, China, Korea, Switzerland and the USA originally signed on to the system arrangement for the VHTR under the Framework Agreement, and Australia joined in 2017. Two project arrangements (PA) were signed within the VHTR system: the Fuel and Fuel Cycle PA and the Hydrogen Production PA.
The HTR-PM demonstration unit in China is under construction at Shidaowan, and will pave the way for a commercial version of the VHTR.
The main research needs, according to the China Academy of Sciences, are fuels, materials and hydrogen production.
Generation IV reactor designs under development by GIF
* high = 7-15 MPa + = with some U-235 or Pu-239 ** 'battery' model with long cassette core life (15-20 yr) or replaceable reactor module.
European programme from 2010
The European Commission in 2010 launched the European Sustainable Nuclear Industrial Initiative ( ESNII ), which will support three Generation IV fast reactor projects as part of the EU’s plan to promote low-carbon energy technologies. Other initiatives supporting biomass, wind, solar, electricity grids and carbon sequestration are in parallel. ESNII was to take forward: the Astrid sodium-cooled fast reactor (SFR) proposed by France for construction there, the Allegro gas-cooled fast reactor (GFR) supported by central and eastern Europe, and the ALFRED lead-cooled fast reactor (LFR) technology pilot in Romania, supported by the MYRRHA lead-bismuth facility in Belgium. Astrid was cancelled in 2019.
The aim of ESNII is to demonstrate Gen IV reactor technologies that can close the nuclear fuel cycle, provide long-term waste management solutions, and expand the applications of nuclear fission beyond electricity production to hydrogen production, industrial heat and desalination. ESNII is designed to combine European capabilities in fast neutron reactor R&D with industrial capability to build the prototypes and develop supporting infrastructure.
The total estimated cost to ESNII of deploying these Gen IV prototypes past 2020 was €10.8 billion: €5 billion for Astrid (cancelled in 2019), €1.96 billion is for ALFRED and MYRRHA, a technology pilot and a later LFR demonstrator, and €1.2 billion for Allegro. Supporting infrastructure is projected to cost €2.65 billion. The 2010-12 ESNII budget was €527 million, including €329 million for Astrid.
Astrid SFR was led by the French CEA, involved EdF and Areva, and was supported by a French government loan of €651 million. Astrid was based on about 45 reactor-years of operational experience in France and would have been rated 400 to 600 MWe. It was expected to be built at Marcoule from 2020, with the unit being connected to the grid in 2025. The project was cancelled in 2019.
Allegro GFR is to be built in eastern Europe, and is more innovative. It is rated at 75 MWt and is being developed out of the EU GoFastR project. The ALLIANCE project (Preparation of Allegro – Implementing Advanced Nuclear Fuel Cycle in Central Europe) was then launched in 2012 to continue the elaboration of basic documents needed for high-level decisions and licencing of Allegro. The main nuclear parameters (power density, burn-up etc. ) would be similar to those of the planned 2400 MWth GFR. The core built up from the initial fuel type (MOX) will be replaced by a core of ceramic fuel for the second half of Allegro operation. The Czech Republic, Hungary and Slovakia made a joint proposal to host the project, with French CEA support. A decision is expected 2025/2026 as to whether the reactor move beyond conceptual study.
In mid-2013 four nuclear research institutes and engineering companies from central Europe’s Visegrád Group of Nations (V4) agreed to establish a centre for joint research, development and innovation in Generation IV nuclear reactors. The V4G4 Centre of Excellence was set up by scientific and research engineering company ÚJV Řež AS of the Czech Republic, the Academy of Sciences Centre for Energy Research of Hungary, Poland’s National Centre for Nuclear Research, and engineering company VUJE AS of Slovakia. It is focused on gas-cooled fast reactors such as Allegro.
ALFRED LFR technology demonstrator – the Advanced Lead Fast Reactor European Demonstrator – of about 300 MWt is seen as a prelude to an industrial demonstration unit of about 300-400 MWe. ALFRED will employ mixed oxide (MOX) fuel, with about 17% plutonium in equilibrium, and able to recycle minor actinides as about 1% of feed. Construction of ALFRED could begin in the early 2020s.
A consortium was set up in December 2013 for ALFRED's construction, comprising Italy's National Agency for New Technologies, Energy and the Environment (ENEA), Ansaldo Nucleare, and Romania's Nuclear Research Institute (Institutul de Cercetari Nucleare, ICN). The group is to be known as the Fostering Alfred Construction (Falcon) consortium, which will be expanded through the participation of further European organizations. The total cost of the project is put at some €1.0 billion. ALFRED will be built at ICN's facility in Mioveni, near Pitesti in southern Romania, where a fuel manufacturing plant is in operation for the country's two operating Candu reactors.
The MYRRHA LFR project is initially a 57 MWt accelerator-driven system with a liquid lead-bismuth (Pb-Bi) spallation target that in turn couples to a Pb-Bi cooled, subcritical fast nuclear core. Later it will become a European fast neutron technology pilot plant for lead and a multi-purpose research reactor. Belgium’s SCK-CEN is leading the project and will provide a total of about €560 million. The unit is rated at 100 thermal MW. It will be built at SCK-CEN’s Mol site. A reduced-power model of MYRRHA called Guinevere started up at Mol in March 2010.
Notes & references
General references.
GIF Annual Reports GIF 2014, Technology roadmap update for Gen IV nuclear energy systems ELSY Project, 2012 ESNII website
Related information
Nuclear Reactions
Article summary & faqs, what is a nuclear reaction.
A nuclear reaction is considered to be the process in which two atomic nuclei or subatomic particles interact to produce one or more new particles or gamma rays .
- Perhaps the most notable nuclear reactions are the nuclear fusion reactions of light elements that power the energy production of stars and the Sun.
- The most notable man-controlled nuclear reaction is the fission reaction which occurs in nuclear reactors .
- In direct nuclear reactions, a projectile and a target nucleus are within the range of nuclear forces for a very short time allowing for an interaction of a single nucleon only .
- In compound nuclear reactions, a projectile and a target nucleus are within the range of nuclear forces for the time allowing for a large number of interactions between nucleons .
- 10B(n,alpha)7Li is typical notation of nuclear reactions.
- Energetics of nuclear reactions is determined by the Q-value of that reaction.
Although the number of possible nuclear reactions is enormous, nuclear reactions can be sorted by types:
- Elastic scattering
- Inelastic scattering
- Capture reactions
- Transfer reactions
- Fission reactions
- Fusion reactions
- Spallation reactions
- Nuclear decay
10B(n, alpha)7Li is a typical nuclear reaction in nuclear reactors. This reaction is mainly used to control nuclear reactors because it absorbs neutrons without fission.
In nuclear physics, the nuclear cross-section of a nucleus is commonly used to characterize the probability that a nuclear reaction will occur. The cross-section is typically denoted σ and measured in units of the area [m 2 ]. The standard unit for measuring a nuclear cross-section is the barn , equal to 10 −28 m² or 10 −24 cm² .
Q is positive. The positive Q reactions are said to be exothermic (or exergic). An exothermic nuclear reaction is a reaction in which there is an increase in the kinetic energy of the products. There is a net release of energy since the kinetic energy of the final state is greater than the kinetic energy of the initial state.
A nuclear reaction is a process when two atomic nuclei or subatomic particles interact to produce one or more new particles or gamma rays . Thus, a nuclear reaction must cause a transformation of at least one nuclide to another. Sometimes if a nucleus interacts with another nucleus or particle without changing the nature of any nuclide, the process is referred to as a nuclear scattering rather than a nuclear reaction. Perhaps the most notable nuclear reactions are the nuclear fusion reactions of light elements that power the energy production of stars and the Sun. Natural nuclear reactions also occur in the interaction between cosmic rays and matter.
The most notable man-controlled nuclear reaction is the fission reaction which occurs in nuclear reactors . Nuclear reactors are devices to initiate and control a nuclear chain reaction , but there are not only artificial devices. The world’s first nuclear reactor operated about two billion years ago. The natural nuclear reactor formed at Oklo in Gabon, Africa, when a uranium-rich mineral deposit became flooded with groundwater that acted as a neutron moderator , and a nuclear chain reaction started. These fission reactions were sustained for hundreds of thousands of years until a chain reaction could be supported no longer. This was confirmed by the existence of isotopes of the fission-product gas xenon and by different ratios of U-235 / U-238 (enrichment of natural uranium).
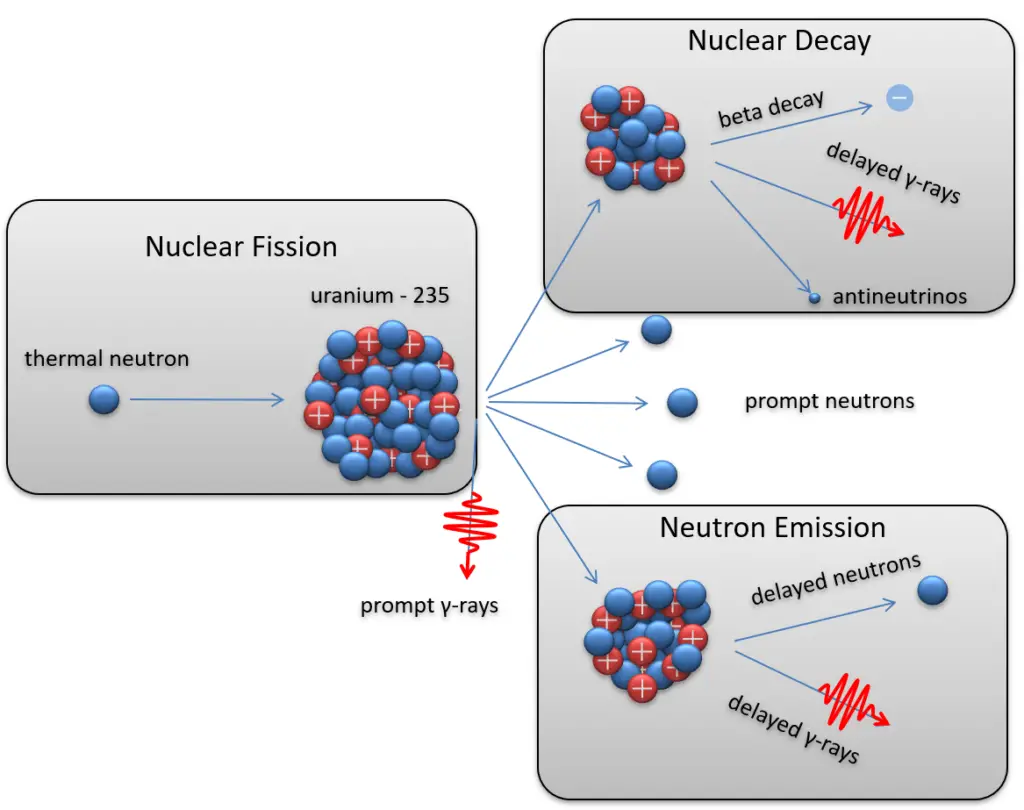
See also: JANIS – Java-based Nuclear Data Information System
See also: Neutron Reactions
Notation of Nuclear Reactions
Standard nuclear notation shows (see picture) the chemical symbol, the mass number, and the atomic number of the isotope.
If the initial nuclei are denoted by a and b , and the product nuclei are denoted by c and d , the reaction can be represented by the equation:
a + b → c + d
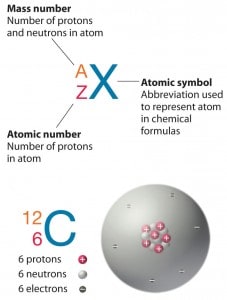
Instead of using the full equations in the style above, a compact notation describes nuclear reactions in many situations. This style of the form a(b,c)d is equivalent to a + b producing c + d . Light particles are often abbreviated in this shorthand, typically p means proton, n means neutron , d means deuteron, α means an alpha particle or helium-4, β means beta particle or electron, γ means gamma photon , etc. The reaction above would be written as 10B(n,α)7Li.
Basic Classification of Nuclear Reactions
To understand the nature of neutron nuclear reactions , the classification according to the time scale of these reactions has to be introduced. Interaction time is critical for defining the reaction mechanism.
There are two extreme scenarios for nuclear reactions (not only neutron reactions):
- A projectile and a target nucleus are within the range of nuclear forces for a very short time allowing for an interaction of a single nucleon only . These types of reactions are called direct reactions .
- A projectile and a target nucleus are within the range of nuclear forces, allowing for many interactions between nucleons . These types of reactions are called the compound nucleus reactions .
In fact, there is always some non-direct (multiple internuclear interaction) component in all reactions, but the direct reactions have this component limited.
- The direct reactions are fast and involve a single-nucleon interaction .
- The interaction time must be very short (~10 -22 s) .
- The direct reactions require incident particle energy larger than ∼ 5 MeV/Ap . (Ap is the atomic mass number of a projectile)
- Incident particles interact on the surface of a target nucleus rather than in the volume of a target nucleus.
- Products of the direct reactions are not distributed isotropically in angle , but they are forward-focused.
- Direct reactions are of importance in measurements of nuclear structure.
- The compound nucleus is a relatively long-lived intermediate state of the particle-target composite system.
- The compound nucleus reactions involve many nucleon-nucleon interactions .
- A large number of collisions between the nucleons leads to a thermal equilibrium inside the compound nucleus.
- The time scale of compound nucleus reactions is 10 -18 s – 10 -16 s .
- The compound nucleus reactions are usually created if the projectile has low energy .
- Incident particles interact in the volume of a target nucleus.
- Products of the compound nucleus reactions are distributed near isotropically in angle (the nucleus loses memory of how it was created – Bohr’s hypothesis of independence ).
- The decay mode of the compound nucleus does not depend on how the compound nucleus is formed .
- Resonances in the cross-section are typical for the compound nucleus reaction.
Types of Nuclear Reactions
Although the number of possible nuclear reactions is enormous, nuclear reactions can be sorted by type. Most nuclear reactions are accompanied by gamma emissions. Some examples are:
- Elastic scattering . In elastic scattering, the kinetic energy of a particle is conserved in the center-of-mass frame, but its direction of propagation is modified. There is no energy transferred into nuclear excitation in an elastic scattering reaction. It is a crucial reaction for neutron moderators in nuclear reactors. To be an effective moderator, the probability of an elastic reaction between the neutron and the nucleus must be high.
1H (n, n) 1H
- Inelastic scattering . In inelastic scattering, the particle is absorbed and then re-emitted. The difference of kinetic energies is saved in an excited nuclide. An inelastic scattering plays an important role in slowing down neutrons, especially at high energies and by heavy nuclei.
238U (n, n’) 238U*
- Capture reaction . The capture reaction is one of the two possible absorption reactions that may occur. Capture reactions result in the loss of a neutron coupled with the production of one or more gamma rays. The resulting nucleus may also undergo a subsequent decay, such as beta decay in this example, which is a very important reaction in nuclear fuel.
238U (n, ˠ) 239U
- Transfer Reaction . Transfer reactions are nuclear reactions in which one or more nucleons are transferred to the other nucleus. Transfer reactions can occur from the projectile to the target; stripping reactions, or from the target to the projectile; pick-up reactions. These reactions are common in particle accelerators and astrophysics.
4He (α, p) 7Li
- Fission reactions . Nuclear fission is a nuclear reaction in which the nucleus of an atom splits into smaller parts (lighter nuclei). The fission process often produces free neutrons and photons (in the form of gamma rays) and releases a large amount of energy.
235U (n, 3n) fission products
- Fusion reactions . Occur when two or more atomic nuclei collide at a very high speed and join to form a new type of atomic nucleus. The fusion reaction of deuterium and tritium is exciting because of its potential of providing energy for the future.
3T (d, n) 4He
- Spallation reactions . Spallation reaction occurs when a particle hits a nucleus with sufficient energy and momentum to knock out several small fragments or smash them into many fragments. Nuclear spallation is one of the processes by which a particle accelerator may be used to produce a beam of neutrons.
209Bi (α,xn)213-xAt
- Alpha radioactivity . Alpha particles consist of two protons and two neutrons bound together into a particle identical to a helium nucleus. Because of its huge mass (more than 7000 times the mass of the beta particle) and its charge, it heavily ionizes material and has a very short range.
- Beta radioactivity . Beta particles are high-energy, high-speed electrons or positrons emitted by specific radioactive nuclei such as potassium-40. The beta particles emitted are a form of ionizing radiation, also known as beta rays. The beta particles have a greater range of penetration than alpha particles but still much less than gamma rays. The production of beta particles is termed beta decay.
- Gamma radioactivity . Gamma rays are electromagnetic radiation of a very high frequency and are therefore high-energy photons. Nuclei decay produces them as they transition from a high-energy state to a lower state known as gamma decay. Most nuclear reactions are accompanied by gamma emissions.
- Neutron emission . Neutron emission is a type of radioactive decay of nuclei containing excess neutrons (especially fission products), in which a neutron is simply ejected from the nucleus. This type of radiation plays a key role in nuclear reactor control because these neutrons are delayed neutrons .
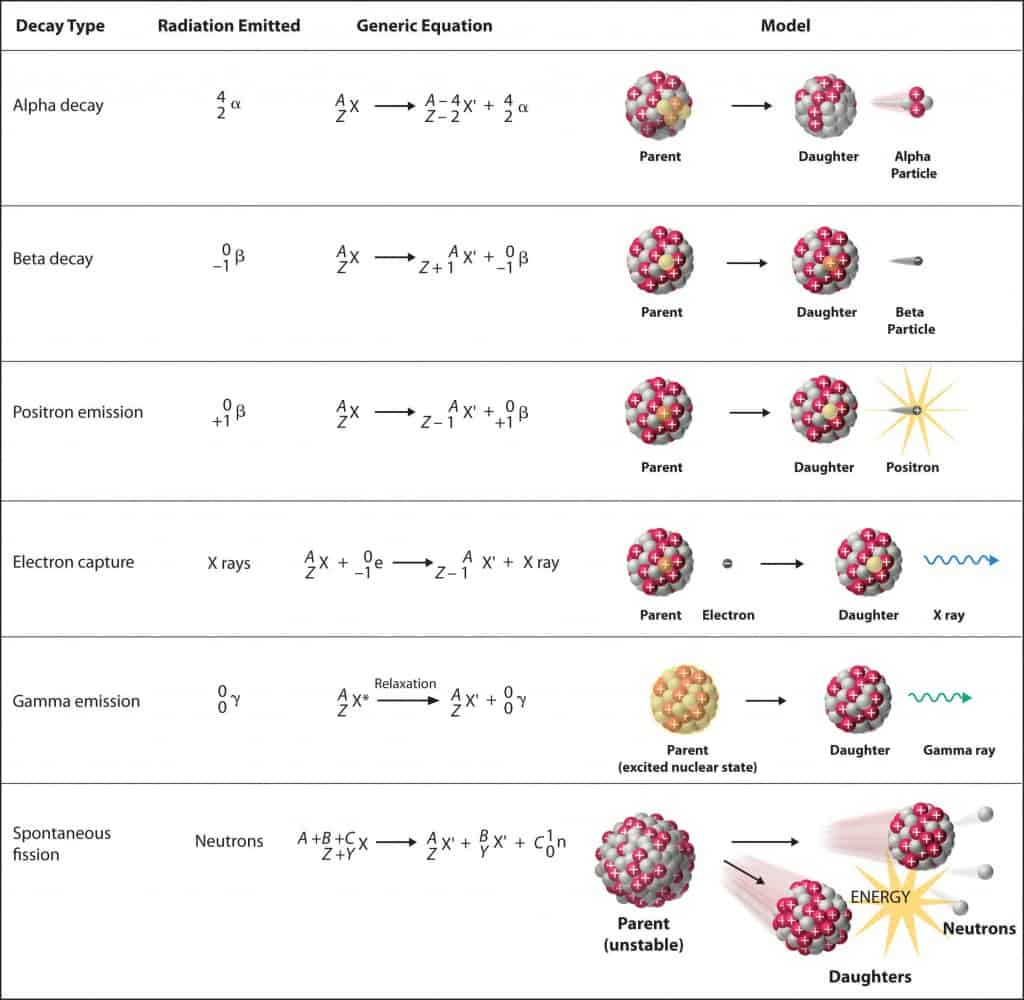
Main Characteristics of Nuclear Reactions
In nuclear physics, the nuclear cross-section of a nucleus is commonly used to characterize the probability that a nuclear reaction will occur. The cross-section is typically denoted σ and measured in units of the area [m 2 ]. The standard unit for measuring a nuclear cross-section is the barn , equal to 10 −28 m² or 10 −24 cm² . It can be seen that the concept of a nuclear cross-section can be quantified physically using a “characteristic target area,” where a larger area means a larger probability of interaction.
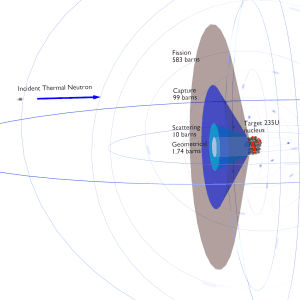
- σ is the cross-section of this event [m 2 ],
- μ is the attenuation coefficient due to the occurrence of this event [m -1 ],
- n is the density of the target particles [m -3 ].
Conservation Laws in Nuclear Reactions
In analyzing nuclear reactions, we apply the many conservation laws . Nuclear reactions are subject to classical conservation laws for the charge, momentum, angular momentum, and energy (including rest energies). Other conservation laws, not anticipated by classical physics, are:
- Law of Conservation of Lepton Number
- Law of Conservation of Baryon Number
- Law of Conservation of Electric Charge
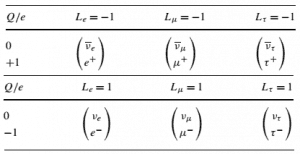
In particle physics , the lepton number is used to denote which particles are leptons and which particles are not. Each lepton has a lepton number of 1, and each antilepton has a lepton number of -1 . Other non-leptonic particles have a lepton number of 0. The lepton number is a conserved quantum number in all particle reactions. A slight asymmetry in the laws of physics allowed leptons to be created in the Big Bang.
The conservation of lepton number means that whenever a lepton of a certain generation is created or destroyed in a reaction, a corresponding antilepton from the same generation must be created or destroyed. It must be added, and there is a separate requirement for each of the three generations of leptons, the electron, muon, and tau, and their associated neutrinos.
Consider the decay of the neutron . The reaction involves only first-generation leptons : electrons and neutrinos :
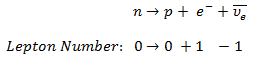
Since the lepton number must be equal to zero on both sides and it was found that the reaction is a three-particle decay ( the electrons emitted in beta decay have a continuous rather than a discrete spectrum ), the third particle must be an electron antineutrino.
In particle physics, the baryon number is used to denote which particles are baryons and which particles are not. Each baryon has a baryon number of 1, and each antibaryon has a baryon number of -1. Other non-baryonic particles have a baryon number of 0. Since there are exotic hadrons like pentaquarks and tetraquarks, there is a general definition of baryon number as:
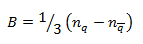
where n q is the number of quarks, and n q is the number of antiquarks.
The baryon number is a conserved quantum number in all particle reactions.
The law of conservation of baryon number states that:
The sum of the baryon number of all incoming particles is the same as the sum of the baryon numbers of all particles resulting from the reaction.
For example, the following reaction has never been observed:
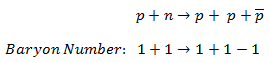
even if the incoming proton has sufficient energy and charge, energy, and so on, are conserved. This reaction does not conserve the baryon number since the left side has B =+2, and the right has B =+1.
On the other hand, the following reaction (proton-antiproton pair production) does conserve B and does occur if the incoming proton has sufficient energy (the threshold energy = 5.6 GeV):

As indicated, B = +2 on both sides of this equation.
From these and other reactions, the conservation of the baryon number has been established as a basic principle of physics.
This principle provides the basis for the stability of the proton . Since the proton is the lightest particle among all baryons, the hypothetical products of its decay would have to be non-baryons. Thus, the decay would violate the conservation of the baryon number. It must be added some theories have suggested that protons are, in fact, unstable with a very long half-life (~10 30 years) and that they decay into leptons. There is currently no experimental evidence that proton decay occurs.
The law of conservation of electric charge can be demonstrated also on positron-electron pair production . Since the gamma-ray is electrically neutral and the sum of the electric charges of electron and positron is also zero, the electric charge in this reaction is also conserved.
Ɣ → e – + e +
It must be added, for electron-positron pair production to occur, the electromagnetic energy of the photon must be above threshold energy , which is equivalent to the rest mass of two electrons. The threshold energy (the total rest mass of produced particles) for electron-positron pair production equals 1.02MeV (2 x 0.511MeV) because the rest mass of a single electron is equivalent to 0.511MeV of energy. If the origin photon’s energy is greater than 1.02MeV, any energy above 1.02MeV is, according to the conservation law, split between the kinetic energy of motion of the two particles. The presence of an electric field of a heavy atom such as lead or uranium is essential to satisfy the conservation of momentum and energy . To satisfy the conservation of momentum and energy, the atomic nucleus must accept some momentum. Therefore a photon pair production in free space cannot occur.
Certain of these laws are obeyed under all circumstances, and others are not. We have accepted the conservation of energy and momentum. In all the examples given, we assume that the number of protons and the number of neutrons is separately conserved. We shall find circumstances and conditions in which this rule is not true. Where we are considering non-relativistic nuclear reactions, it is essentially true. However, we shall find that these principles must be extended when we consider relativistic nuclear energies or those involving weak interactions.
Some conservation principles have arisen from theoretical considerations, and others are just empirical relationships. Notwithstanding, any reaction not expressly forbidden by the conservation laws will generally occur, if perhaps at a slow rate. This expectation is based on quantum mechanics. Unless the barrier between the initial and final states is infinitely high, there is always a non-zero probability that a system will make the transition between them.
The non-relativistic reactions are governing by four of the fundamental laws and to analyze them is necessary to follow them:
- Conservation of nucleons . The total number of nucleons before and after a reaction are the same.
- Conservation of charge . The sum of the charges on all the particles before and after a reaction are the same.
- Conservation of momentum . The total momentum of the interacting particles before and after a reaction is the same.
- Conservation of energy . Energy, including rest mass energy, is conserved in nuclear reactions.
Reference: Lamarsh, John R. Introduction to Nuclear engineering 2nd Edition.
Elastic Nuclear Collision
A neutron (n) of mass 1.01 u traveling with a speed of 3.60 x 10 4 m/s interacts with a carbon (C) nucleus ( m C = 12.00 u ) initially at rest in an elastic head-on collision .
What are the velocities of the neutron and carbon nucleus after the collision?
This is an elastic head-on collision of two objects with unequal masses . We have to use the conservation laws of momentum and kinetic energy and apply them to our system of two particles.
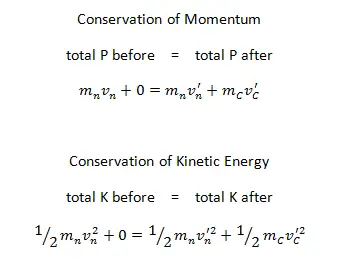
We can solve this equation system, or we can use the equation derived in the previous section. This equation stated that the relative speed of the two objects after the collision has the same magnitude (but opposite direction) as before the collision, no matter what the masses are.
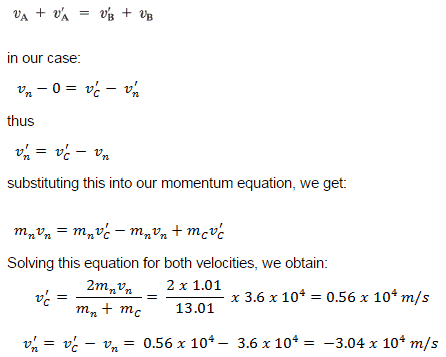
The minus sign for v’ tells us that the neutron scatters the back of the carbon nucleus because the carbon nucleus is significantly heavier. On the other hand, its speed is less than its initial speed. This process is known as neutron moderation, and it significantly depends on the mass of moderator nuclei.
Energetics of Nuclear Reactions – Q-value
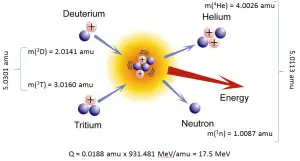
In nuclear and particle physics, the energetics of nuclear reactions are determined by the reaction’s Q-value. The Q-value of the reaction is defined as the difference between the sum of the masses of the initial reactants and the sum of the masses of the final products in energy units (usually in MeV).
Consider a typical reaction in which the projectile a and target A place to two products, B and b. This can also be expressed in the notation we have used so far, a + A → B + b , or even in a more compact notation, A(a,b)B .
See also: E=mc 2
The Q-value of this reaction is given by:
Q = [m a + m A – (m b + m B )]c 2
which is the same as the excess kinetic energy of the final products:
Q = T final – T initial
= T b + T B – (T a + T A )
For reactions in which there is an increase in the kinetic energy of the products, Q is positive . The positive Q reactions are said to be exothermic (or exergic ). There is a net release of energy since the kinetic energy of the final state is greater than the kinetic energy of the initial state.
For reactions in which there is a decrease in the kinetic energy of the product, Q is negative . The negative Q reactions are endothermic (or endoergic ), and they require net energy input.
The energy released in a nuclear reaction can appear mainly in one of three ways:
- The kinetic energy of the products.
- Emission of gamma rays . Gamma rays are emitted by unstable nuclei in their transition from a high-energy state to a lower state known as gamma decay.
- Metastable state . Some energy may remain in the nucleus as a metastable energy level.
A small amount of energy may also emerge in the form of X-rays. Generally, products of nuclear reactions may have different atomic numbers, and thus the configuration of their electron shells is different in comparison with reactants. As the electrons rearrange themselves and drop to lower energy levels, internal transition X-rays (X-rays with precisely defined emission lines) may be emitted.
Exothermic Reactions
The DT fusion reaction of deuterium and tritium is particularly interesting because of its potential to provide future energy. Calculate the reaction Q-value .
The atom masses of the reactants and products are:
m( 3 T) = 3.0160 amu
m( 2 D) = 2.0141 amu
m( 1 n) = 1.0087 amu
m( 4 He) = 4.0026 amu
Using the mass-energy equivalence , we get the Q-value of this reaction as:
Q = {(3.0160+2.0141) [amu] – (1.0087+4.0026) [amu]} x 931.481 [MeV/amu]
= 0.0188 x 931.481 = 17.5 MeV
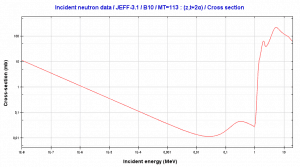
Tritium is a byproduct of nuclear reactors . Most of the tritium produced in nuclear power plants stems from boric acid , commonly used as a chemical shim to compensate for an excess of initial reactivity. The main reaction in which the tritium is generated from boron is below:
10B(n,2*alpha)T
This neutron reaction with an isotope 10 B is the main way radioactive tritium in the primary circuit of all PWRs is generated. Note that this reaction is a threshold reaction due to its cross-section.
Calculate the reaction Q-value .
m( 10 B) = 10.01294 amu
m( 1 n) = 1.00866 amu
m( 3 T) = 3.01604 amu
Q = {(10.0129+1.00866) [amu] – (3.01604+2 x 4.0026) [amu]} x 931.481 [MeV/amu]
= 0.00036 x 931.481 = 0.335 MeV
Endothermic Reactions
A high-energy photon ( gamma-ray ) can, under certain conditions, eject a neutron from a nucleus. It occurs when its energy exceeds the binding energy of the neutron in the nucleus. Most nuclei have binding energies over 6 MeV , above the energy of most gamma rays from fission . On the other hand, few nuclei with sufficiently low binding energy are of practical interest . These are 2 D, 9 Be , 6 Li, 7 Li, and 13 C. As can be seen from the table, the lowest threshold has 9 Be with 1.666 MeV and 2 D with 2.226 MeV .
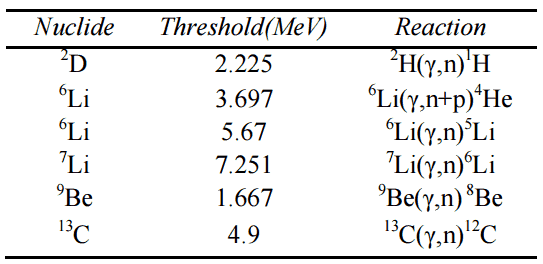
In the case of deuterium, neutrons can be produced by the interaction of gamma rays (with a minimum energy of 2.22 MeV) with deuterium:
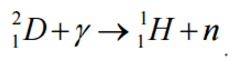
The reaction Q-value is calculated below:
The atom masses of the reactant and products are:
m( 2 D) = 2.01363 amu
m( 1 H) = 1.00728 amu
Q = {2.01363 [amu] – (1.00866+1.00728) [amu]} x 931.481 [MeV/amu]
= -0.00231 x 931.481 = -2.15 MeV
7Li (α, n) 10B
m( 7 Li) = 7.0160 amu
Using the m ass-energy equivalence , we get the Q-value of this reaction as:
Q = {(7.0160+4.0026) [amu] – (1.0087+10.01294) [amu]} x 931.481 [MeV/amu]
= 0.00304 x 931.481 = -2.83 MeV
Test your Knowledge – Nuclear Reactions
With our simple quizzes, you can test your knowledge.
It is intuitive: start quiz and answer questions.
- J. R. Lamarsh, Introduction to Nuclear Reactor Theory, 2nd ed., Addison-Wesley, Reading, MA (1983).
- J. R. Lamarsh, A. J. Baratta, Introduction to Nuclear Engineering, 3d ed., Prentice-Hall, 2001, ISBN: 0-201-82498-1.
- W. M. Stacey, Nuclear Reactor Physics, John Wiley & Sons, 2001, ISBN: 0- 471-39127-1.
- Glasstone, Sesonske. Nuclear Reactor Engineering: Reactor Systems Engineering, Springer; 4th edition, 1994, ISBN: 978-0412985317
- W.S.C. Williams. Nuclear and Particle Physics. Clarendon Press; 1 edition, 1991, ISBN: 978-0198520467
- Kenneth S. Krane. Introductory Nuclear Physics, 3rd Edition, Wiley, 1987, ISBN: 978-0471805533
- G.R.Keepin. Physics of Nuclear Kinetics. Addison-Wesley Pub. Co; 1st edition, 1965
- Robert Reed Burn, Introduction to Nuclear Reactor Operation, 1988.
- U.S. Department of Energy, Nuclear Physics and Reactor Theory. DOE Fundamentals Handbook, Volume 1 and 2. January 1993.
Advanced Reactor Physics:
- K. O. Ott, W. A. Bezella, Introductory Nuclear Reactor Statics, American Nuclear Society, Revised edition (1989), 1989, ISBN: 0-894-48033-2.
- K. O. Ott, R. J. Neuhold, Introductory Nuclear Reactor Dynamics, American Nuclear Society, 1985, ISBN: 0-894-48029-4.
- D. L. Hetrick, Dynamics of Nuclear Reactors, American Nuclear Society, 1993, ISBN: 0-894-48453-2.
- E. E. Lewis, W. F. Miller, Computational Methods of Neutron Transport, American Nuclear Society, 1993, ISBN: 0-894-48452-4.
See previous:
Radioactive Decay
Atomic and Nuclear Physics
Binding Energy

COMMENTS
In nuclear reactors, neutrons travel with energies of 5 × 10-21J. Find their speed and wavelength. Maharashtra State Board HSC Science ... Important Solutions 5194. Concept Notes & Videos 416. Time Tables 27. Syllabus. In nuclear reactors, neutrons travel with energies of 5 × 10-21J. Find their speed and wavelength. - Physics. Advertisements.
Figure 21.4.5 21.4. 5: (a) In a subcritical mass, the fissile material is too small and allows too many neutrons to escape the material, so a chain reaction does not occur. (b) In a critical mass, a large enough number of neutrons in the fissile material induce fission to create a chain reaction.
The absorption cross-section is often highly dependent on neutron energy. Note that nuclear fission produces neutrons with a mean energy of 2 MeV (200 TJ/kg, i.e., 20,000 km/s). The neutron can be roughly divided into three energy ranges: Fast neutron. (10MeV - 1keV) Resonance neutron (1keV - 1eV) Thermal neutron. (1eV - 0.025eV)
Energy Deposition by Neutrons. Neutrons are generated over a wide range of energies by a variety of different processes. Like photons, neutrons are uncharged and do not interact with orbital electrons. Neutrons can travel considerable distances through matter without interacting. Neutrons will interact with atomic nuclei through several mechanisms.
In a nuclear reactor neutrons can have energies ranging from 10-3ev (1 mev) to 107 ev (10 Mev). This means our study of neutron interactions, in principle, will have to cover an energy range of 10 ten orders of magnitude. In practice we will limit ourselves to two energy ranges, the slowing down region (ev to Kev) and the thermal region (around ...
Describe briefly the type of reaction on which a nuclear fission reactor operates. • In nuclear fission a heavy nucleus disassociates into two medium nuclei. In a reactor the fission is induced. It takes place after a heavy nucleus captures a neutron. For example. 235. U→X+ Yn · ·· Why is energy released, and roughly how much per ...
The Basic Physics of Neutron Transport. Let us consider a neutron, streaming with direction Ω and energy E inside a material with known physical properties. As the neutron travels an incremental distance ds, there is an incremental probability dp that the neutron will interact with a nucleus. To determine the relationship between dp and ds, let us consider the neutron to be normally incident ...
The physics of a nuclear reactor is governed by the flux distribution profile of the neutrons in the system with respect to space, energy, and time. The neutron transport is, thus, a complex phenomenon and is required to be tracked with respect to each of these quantities. The governing equation for this behavior is the neutron transport equation.
The Neutron Energy Spectrum in a Nuclear Reactor A knowledge of the physical theory of nuclear reactors is necessary in ... and all the neutrons are traveling in the same direction with a common speed v, the neutron flux at any point in the beam is defined as 4> = nv (3.1)
Introduction. Nuclear fission is the process in which the nucleus of an atom is split, forming nuclei of lighter atoms and neutrons. The mass of these products is less than the original mass. According to Einstein's equation \(E=mc^2\), the small amount of missing mass is converted into a large amount of energy.
The Science. Nuclear fission and fusion reactors use the elements carbon and silicon as shielding and structural materials. Nuclear engineers also use these elements in fuel and in neutron moderators, which control the speed of neutrons to help maintain chain reactions. Silicon carbide, for example, can be used to clad fuel and as a pellet ...
Modern nuclear reactors may contain as many as 10 million fuel pellets. The amount of energy in each of these pellets is equal to that in almost a ton of coal or 150 gallons of oil. Nuclear Moderators. Neutrons produced by nuclear reactions move too fast to cause fission (refer back to Figure 21.17). They must first be slowed to be absorbed by ...
A pressurized water reactor (PWR) is a common design of a nuclear reactor. In a PWR, water functions as a coolant and as a moderator. A moderator slows down the neutrons, because slower moving neutrons are better at causing fission to occur. A moderator is usually water; however, graphite and heavy water can also be used.
The neutrons can be roughly (for purposes of reactor physics) divided into three energy ranges: Thermal neutrons (0.025 eV - 1 eV). Resonance neutrons (1 eV - 1 keV). Fast neutrons (1 keV - 10 MeV). Even most reactor computing codes use only two neutron energy groups: Slow neutrons group (0.025 eV - 1 keV).
Nuclear reactor - Thermal, Intermediate, Fast: Reactors are conveniently classified according to the typical energies of the neutrons that cause fission. Neutrons emanating in fission are very energetic; their average energy is around two million electron volts (MeV), nearly 80 million times the energy of atoms in ordinary matter at room temperature. As neutrons scatter or collide with nuclei ...
In this case, the target must be bombarded with accelerated hydrogen (H), deuterium (D), or tritium (T) nuclei. Neutron Generators. Neutrons are produced in the fusion of deuterium and tritium in the following exothermic reaction. 2D + 3T → 4He + n + 17.6 MeV. The neutron is produced with a kinetic energy of 14.1 MeV.
The transfer of neutron's energy to the absorbing medium is much less efficient when m n M; the larger is M, the less efficient is the energy transfer, as evident from(9.2).Forexample,asshowninSect.5.3.4,(9.2)predictsanonly2%fractional energy transfer from a neutron colliding head-on with a lead nucleus, compared to
The neutron energies are deduced from the measured recoil energies of the protons during the deceleration of the neutrons in paraffin. ... {\text{UO}_{3}}}\)) is irradiated by thermal neutrons from a nuclear reactor. The two fragments produced during the nuclear fission fly into opposite directions (conservation of momentum).
Description: The full, seven-dimensional neutron transport equation is developed from physical intuition, and putting that intuition into math. Aspects of neutron creation and transport are introduced as needed—neutron energy birth spectrum, flux, current, and many different types of neutron cross sections (fission, capture, scattering, total).
Dmitry Terentyev is a Fusion R&D Programme Manager at the Belgian Nuclear Research Centre known as SCK CEN. He and his team are receiving materials through the IAEA project and blasting them at their neutron source facility in Mol. In February, they received their first batch. "We are using the fission research reactor 'BR2' as a neutron ...
The 235 U fission cross-section (from the EXFOR database) is σ fiss (E) = 585.1 b at 25 meV neutron energy (that is, the thermal energy), where the neutron energy spectrum of the typical thermal ...
A combination of radiochemistry and radiation chemistry is used to study nuclear reactions such as fission and fusion. Some early evidence for nuclear fission was the formation of a short-lived radioisotope of barium which was isolated from neutron irradiated uranium (139 Ba, with a half-life of 83 minutes and 140 Ba, with a half-life of 12.8 days, are major fission products of uranium).
Nuclear Fuel and its Fabrication. Updated Wednesday, 13 October 2021. Fuel fabrication is the last step in the process of turning uranium into nuclear fuel rods. Batched into assemblies, the fuel rods form the majority of a reactor core's structure. This transformation from a fungible material - uranium - to high-tech reactor components is ...
Here are some common terms that may cause confusion about the nation's largest source of clean power: 1. Critical. "Criticality" might sound as serious as a heart attack, but in the nuclear energy world it's a reason to jump for joy. We say a nuclear reactor is "critical" when it is perfectly stable.
Updated Thursday, 1 April 2021. Improved designs of nuclear power reactors are constantly being developed internationally. The first so-called Generation III advanced reactors have been operating in Japan since 1996. These have now evolved further. Newer advanced reactors now being built have simpler designs which are intended to reduce capital ...
The neutron absorption reaction is the most important type of reactions that take place in a nuclear reactor.The absorption reactions are reactions where the neutron is completely absorbed, and the compound nucleus is formed.This is a very important feature because the mode of decay of such a compound nucleus does not depend on how the compound nucleus was formed.
Generation IV Nuclear Reactors. Updated Tuesday, 30 April 2024. An international task force is sharing R&D to develop six generation IV nuclear reactor technologies. Four are fast neutron reactors. All of these operate at higher temperatures than today's reactors. In particular, four are designated for hydrogen production.
Nuclear power was back in the global spotlight last week at the 26th World Energy Congress as an IAEA delegation led by Director General Rafael Mariano Grossi highlighted its key role on energy security and climate change. The world's longest-running energy event is the flagship event of the World Energy Council, the oldest independent energy ...
Nuclear fission is a nuclear reaction in which the nucleus of an atom splits into smaller parts (lighter nuclei). The fission process often produces free neutrons and photons (in the form of gamma rays) and releases a large amount of energy. 235U (n, 3n) fission products. Fusion reactions.
CERN Scientists Break Silence On What Just Emerged Inside The Premises