Majid Safari
Affiliation, publication topics, ieee account.
- Change Username/Password
- Update Address

Purchase Details
- Payment Options
- Order History
- View Purchased Documents
Profile Information
- Communications Preferences
- Profession and Education
- Technical Interests
- US & Canada: +1 800 678 4333
- Worldwide: +1 732 981 0060
- Contact & Support
- About IEEE Xplore
- Accessibility
- Terms of Use
- Nondiscrimination Policy
- Privacy & Opting Out of Cookies
A not-for-profit organization, IEEE is the world's largest technical professional organization dedicated to advancing technology for the benefit of humanity. © Copyright 2024 IEEE - All rights reserved. Use of this web site signifies your agreement to the terms and conditions.
Sitaatteja vuodessa
Päällekkäiset lähteet, yhdistetyt sitaatit, lisää muut kirjoittajat muut kirjoittajat, viittaukset näytä kaikki, muut kirjoittajat.
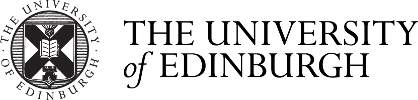
School of Engineering
> schools & departments, search form.
SCHOOL OF ENGINEERING
Electronics and Electrical Engineering People
All people working in Electronics and Electrical Engineering.
You can also use our Search form or browse all people working at the School of Engineering .

THE UNIVERSITY OF EDINBURGH
- Terms & Conditions
- Privacy Notice
- Privacy & Cookies
- Website Accessibility
- Freedom of information publication scheme
- Website Issue Reporting

- Social Media

- Schools & departments

Find your exchange coordinator
All Schools and Subject Areas offering exchange opportunities have an Exchange Coordinator to support and advise students before and during their exchange.
The Exchange Coordinator is a member of academic staff who will be a key contact throughout your exchange. Many Coordinators hold information sessions at the start of the academic year to inform students of the opportunities available in their subject area.
Arts, Humanities and Social Science
Science and engineering, medicine and veterinary medicine.
Open Access is an initiative that aims to make scientific research freely available to all. To date our community has made over 100 million downloads. It’s based on principles of collaboration, unobstructed discovery, and, most importantly, scientific progression. As PhD students, we found it difficult to access the research we needed, so we decided to create a new Open Access publisher that levels the playing field for scientists across the world. How? By making research easy to access, and puts the academic needs of the researchers before the business interests of publishers.
We are a community of more than 103,000 authors and editors from 3,291 institutions spanning 160 countries, including Nobel Prize winners and some of the world’s most-cited researchers. Publishing on IntechOpen allows authors to earn citations and find new collaborators, meaning more people see your work not only from your own field of study, but from other related fields too.
Brief introduction to this section that descibes Open Access especially from an IntechOpen perspective
Want to get in touch? Contact our London head office or media team here
Our team is growing all the time, so we’re always on the lookout for smart people who want to help us reshape the world of scientific publishing.
Home > Authors and editors
Majid Safari
1 chapters authored
Chapters authored
By Ahmad Adnan Qidan, Mohammad Dehghani Soltani, Wajahat Ali, Rui Chen, Shenjie Huang, Barzan Yosuf, Sanaa Mohamed, Ravinder Singh, Yi Liu, Hossein Kazemi, Elham Sarbazi, Bela Berde, Dominique Chiaroni, Bastien Bechadergue, Fathi Abdeldayem, Hardik Soni, Jose Tabu, Micheline Perrufel, Nikola Serafimovski, Taisir E.H. El-Gorashi, Michael Crisp, Richard Penty, Ian H. White, Harald Haas, Majid Safari and Jaafar Elmirghani
Optical wireless communication (OWC) is a strong candidate in the sixth generation (6G) of wireless communications as it can support data transmission at high communication speeds, low power consumption, high security, and high reliability. Near-infrared lasers, specifically vertical cavity surface emitting lasers (VCSELs), exhibit larger modulation bandwidth compared to light-emitting diodes (LEDs), and can provide aggregate data rates ranging up to Terabit per second (Tbps). This chapter proposes innovative optical transmitter and receiver designs for indoor laser-based wireless communications, aiming to provide seamless coverage in high-speed multi-user scenarios. The proposed optical transmitter is based on a 5 × 5 VCSEL array where each VCSEL can provide 10 Gigabit per second (Gbps) in a uniform coverage area of 20 cm x 20 cm, achieving a total of 250 Gbps in an area of 1 m 2 at 3 m transmission distance. Moreover, two wide-FOV receiver designs that employ photodetector (PD) arrays in along with imaging or non-imaging optics are introduced to support such an ultra-high-speed optical link. The new design of OWC requires potential techniques to address various networking challenges, including cell formation, interference management, resource allocation, and backhauling. To this end, potential solutions for these challenges are discussed to help establish an end-to-end OWC infrastructure for practical deployment.
Part of the book: Free Space Optics Technologies in B5G and 6G Era - Recent Advances, New Perspectives and Applications [Working title]
Related collaborators
Dominique chiaroni, mazen abdullatif.
University of Aleppo , Syria
Ahmad Qidan
Jaafar elmirghani.
King's college London
Mohammad Dehghani Soltani
The University of Edinburgh
Wajahat Ali
The university of Cambridge
The University of Cambridge
Shenjie Huang
Barzan yosuf.
The University of Leeds
Sanaa Mohamed
King's College London , United Kingdom
Interference Mitigation using Optimized Angle Diversity Receiver in LiFi Cellular network
Light-fidelity (LiFi) is an emerging technology for high-speed short-range mobile communications. Inter-cell interference (ICI) is an important issue that limits the system performance in an optical attocell network. Angle diversity receivers (ADRs) have been proposed to mitigate ICI. In this paper, the structure of pyramid receivers (PRs) and truncated pyramid receivers (TPRs) are studied. The coverage problems of PRs and TPRs are defined and investigated, and the lower bound of field of view (FOV) for each PD is given analytically. The impact of random device orientation and diffuse link signal propagation are taken into consideration. The performances of PRs and TPRs are compared and then optimized ADR structures are proposed. The performance comparison between the select best combining (SBC) and maximum ratio combining (MRC) is given under different noise levels. It is shown that SBC will outperform MRC in an interference limited system, otherwise, MRC is a preferred scheme. In addition, the double source system, where each LiFi AP consists of two sources transmitting the same information signals but with opposite polarity, is proved to outperform the single source (SS) system under certain conditions.
I Introduction
Due to the increasing demand for wireless data, the radio frequency (RF) spectrum has become a very limited resource. Alternative approaches are investigated to support future growth in data traffic and next-generation high-speed wireless communication systems. Techniques such as massive multiple-input multiple-output (MIMO), millimeter wave (mmWave) communications and Light-Fidelity (LiFi) are being explored. Among these technologies, LiFi is a novel bi-directional, high-speed and fully networked wireless communication technology. A typical LiFi system uses off-the-shelf low-cost commercially available light emitting diodes (LEDs) and photodiodes (PDs) as front end devices [ 1 ] . Intensity modulation (IM) is used to encode the information in visible light communication (VLC) since the LED is an incoherent optical source. Direct detection (DD) is adopted at the receiver end. LiFi utilizes visible light as the propagation medium in the downlink for both illumination and communication purposes. It may use infrared light in the uplink in order to allow the illumination constraint of the room to be unaffected, and also to avoid interference with the visible light in the downlink [ 2 ] . The overall license-free bandwidth of visible light is more than 1000 1000 1000 times greater than the whole RF spectrum [ 2 ] . Also, LiFi can provide enhanced security as the light does not penetrate through opaque objects [ 3 ] . In many large indoor environments, multiple light fixtures are installed, these luminaries can act as VLC access points (APs). A network consisting of multiple VLC APs is referred to as a LiFi attocell network [ 2 ] . Given the widespread use of LED lighting, a LiFi attocell network can use the existing lighting infrastructures to offer fully networked wireless connectivity. Moreover, LiFi attocells can be regarded as an additional network layer within the existing wireless networks because there is no interference to the RF counterparts such as femtocell networks [ 2 ] . These benefits of LiFi have made it favorable for recent and future research.
By improving the spatial reuse of the spectrum resources, cellular networks can achieve a higher area spectral efficiency [ 4 ] . In comparison with RF femtocell networks, LiFi attocell networks use smaller cell sizes as the light beams from LEDs are intrinsically narrow [ 5 ] . Thus, with the densely deployed optical APs, the LiFi attocell network can achieve a better bandwidth reuse and a higher area spectral efficiency. However, similar to other cellular systems, inter-cell interference (ICI) in LiFi attocell networks limits the system performance. This is because the signal transmitted to a user will interfere with other users who are receiving signals from the same frequency resource. Particularly, cell-edge users suffer from severe ICI. Despite the dense deployment of APs, due to ICI, LiFi may not provide a uniform coverage concerning data rate. Interference coordination mechanisms have been extensively investigated for VLC systems [ 6 , 7 , 8 , 9 , 10 ] . The commonly used technique is static resource partitioning [ 6 ] . By separating any two cells that reuse the same frequency resource with a minimum reuse distance, ICI is effectively mitigated. However, there is a significant loss in spectral efficiency. A combined wavelength division and code division multiple access scheme was proposed in [ 7 ] . Although this approach enhances the system bandwidth, it requires separate filters for each color band and thus creates additional cost. In [ 8 ] , the fractional frequency reuse (FFR) technique is proposed to mitigate ICI. The FFR scheme is a cost-effective approach to provide improvements both in cell-edge user performance and average spectral efficiency, but a low user-density will decrease the average spectral efficiency significantly. Joint transmission (JT) has been proven to improve signal quality for cell-edge users [ 9 ] . The downside of the JT systems is the extra signaling overhead. Moreover, the space division multiple access (SDMA) scheme using angle diversity transmitters proposed in [ 10 ] can mitigate ICI by generating concentrated beams to users at different locations.
The angle diversity reception, first proposed in [ 11 ] , allows the receiver to achieve a wide field of view (FOV) and high optical gain simultaneously. An angle diversity receiver (ADR) is composed of multiple narrow-FOV PDs facing in different directions. In [ 12 , 13 , 14 , 15 , 16 , 17 , 18 , 19 ] , the ADR is used to address the issue of ICI as well as frequency reuse in LiFi cellular systems, and different signal combining schemes are investigated. However, the proposed ADR structure is hard to implement and the optimum ADR design is not given. Moreover, the system is assumed to be interference limited instead of noise limited in [ 15 ] , which is not always true as the ADR can mitigate most of ICI with noise being the dominated part. Recently, due to the lower channel correlation achieved from the angle diversity scheme, ADRs are introduced to improve the performance of indoor MIMO-VLC systems, and the pyramid receivers (PRs) are proposed [ 20 ] . The generalized structure of truncated pyramid receivers (TPRs) are given in [ 21 ] to reduce the signal to interference plus noise ratio (SINR) fluctuation. However, the optimum structures of the ADRs are not given and therefore the performance gain is not fully exploited. In addition, to obtain a more accurate evaluation of the system performance, the following three factors must be taken into consideration:
I- 1 User Device Orientation
Most of the studies on ADRs assume that the receiving device is pointed vertically upward. However, it has been shown in our previous works that the random orientation of mobile devices can significantly affect the direct current (DC) channel gain and thus the system performance [ 22 , 23 ] . Therefore, the random orientation of the user equipment (UE) needs to be considered. A random device orientation model has been proposed in [ 22 ] . This model will be applied in this study to evaluate the system more accurately.
I- 2 Diffuse Link Signal Propagation
The non-line-of-sight (NLOS) link is neglected in most LiFi and VLC studies and only the line-of-sight (LOS) channel is considered [ 4 , 5 , 6 , 7 , 8 , 9 ] . In [ 24 ] , it is shown that the LOS link is the dominate link and the effect of the reflected signal can be neglected. However, the UE is assumed to be positioned vertically upward, which is not realistic for mobile devices. In our study, we consider the effect of reflection when random device orientation is applied and the results show that the diffuse link cannot be ignored. A microscopic frequency-domain method for the simulation of the indoor VLC channel is presented in [ 25 ] . A closed form for the transfer function that contains all reflection orders is formulated. The method can be extended to multi-spot transmission without a significant increase in the computational complexity. Therefore, in this study, we will use the frequency-domain method to simulate the impact of the diffuse link.
I- 3 Noise Power Spectral Density
The noise power spectral density of the PD has a huge impact on the analyses of system performance. For different levels of noise power spectral density, the system could be noise-limited, interference-limited or noise-plus-interference limited, which could affect the choices of the signal combing schemes and the cell configurations.
The main contributions of this paper are summarized as follows:
The coverage area of ADRs is defined to differentiate from the coverage area of APs. Analytical expressions for the coverage area of both PRs and TPRs are given.
Based on the constraint set by the coverage area of ADRs, the lower bound of FOV of PDs on an ADR is given for the single source (SS) system. The performance of PRs and TPRs are compared, and optimized ADR structures are proposed to fully exploit the performance gain of ADRs. In addition, the joint effect of the receiver and transmitter bandwidth on the average data rate are analyzed.
The performance comparison between the select best combining (SBC) and maximum ratio combining (MRC) are given regarding different levels of noise power spectral density. It is the first time shown that under certain circumstances, the SBC can outperform the MRC.
The double source (DS) cell system is considered to further mitigate the NLOS interference. The lower bound of FOV of PDs on an ADR is derived and the optimized ADR structures are proposed for the DS system.
By comparing the average SINR between the DS system and the SS system under different levels of noise power spectral density, we present that, in a noise-dominated scenario, the SS system should be applied, otherwise, the DS system is preferred.
The rest of this paper is organized as follows. The system model is introduced in Section \@slowromancap ii @ \@slowromancap ii @ \rm{\@slowromancap ii@} . The generalized structures of ADRs are given in Section \@slowromancap iii @ \@slowromancap iii @ \rm{\@slowromancap iii@} . Section \@slowromancap iv @ \@slowromancap iv @ \rm{\@slowromancap iv@} presents the optimum FOV for PRs and TPRs. The concepts of the optical double-source cell are proposed in Section \@slowromancap v @ \@slowromancap v @ \rm{\@slowromancap v@} . The simulation results and discussions are presented in Section \@slowromancap vi @ \@slowromancap vi @ \rm{\@slowromancap vi@} . Finally, conclusions are drawn in Section \@slowromancap vii @ \@slowromancap vii @ \rm{\@slowromancap vii@} .
II System Model
Ii-a light propagation model.
In indoor optical communications, the signal propagation consists of two components: the LOS link and the diffuse link. Therefore, the overall channel DC gain is the sum of the LOS and diffuse components:
where H LOS subscript 𝐻 LOS H_{\rm{LOS}} represents the LOS DC channel gain between the transmitter (Tx) and receiver (Rx), and H diffuse subscript 𝐻 diffuse H_{\rm{diffuse}} is the diffuse DC channel gain which is the superposition of all NLOS components that are caused by reflections from the surfaces of the walls.
II-A 1 LOS link
It is typically assumed that the LED follows the Lambertian radiation pattern [ 11 ] . The LOS DC gain is thus given by [ 25 ] :
II-A 2 NLOS link
Ii-a 3 the total diffuse dc channel gain.
According to [ 25 ] , the total diffuse DC channel gain with infinite reflection can be calculated by the matrix product:
where 𝐈 𝐈 \mathbf{I} denotes the unity matrix.
II-B Signal Combining Schemes for ADR
An indoor LiFi network is studied and it is assumed that the total number of UE and LiFi APs are N UE subscript 𝑁 UE N_{\rm{UE}} and N L subscript 𝑁 L N_{\rm{L}} , respectively. The set of APs is denoted by 𝒜 = { α ∣ α ∈ [ 1 , N L ] } 𝒜 conditional-set 𝛼 𝛼 1 subscript 𝑁 L \mathcal{A}=\{\alpha\mid\alpha\in[1,N_{\rm{L}}]\} . The set of users is denoted as 𝒰 = { μ ∣ μ ∈ [ 1 , N UE ] } 𝒰 conditional-set 𝜇 𝜇 1 subscript 𝑁 UE \mathcal{U}=\{\mu\mid\mu\in[1,N_{\rm{UE}}]\} . The ADR is used as the Rx and the set of PDs on an ADR is denoted as 𝒫 = { p ∣ p ∈ [ 1 , N PD ] } 𝒫 conditional-set 𝑝 𝑝 1 subscript 𝑁 PD \mathcal{P}=\{p\mid p\in[1,N_{\rm{PD}}]\} , where N PD subscript 𝑁 PD N_{\rm{PD}} denotes the total number of PDs on the ADR. In order to achieve high data rates, the direct current biased optical (DCO)-OFDM is used in this study. The number of OFDM subcarriers is denoted as M 𝑀 M , where M 𝑀 M is an even and positive integer, and the sequence number of OFDM subcarriers is denoted by m ∈ { 0 , 1 , … , M − 1 } 𝑚 0 1 … 𝑀 1 m\in\{0,1,\dots,M-1\} . Two constraints should be satisfied to ensure real and positive signals: i) X ( 0 ) = X ( M / 2 ) = 0 𝑋 0 𝑋 𝑀 2 0 X(0)=X(M/2)=0 , and ii) the Hermitian symmetry constraint, i.e., X ( m ) = X ∗ ( M − m ) 𝑋 𝑚 superscript 𝑋 𝑀 𝑚 X(m)=X^{*}(M-m) , for m ≠ 0 𝑚 0 m\neq 0 , where ( ⋅ ) ∗ superscript ⋅ (\cdot)^{*} denotes the complex conjugate operator [ 26 ] . Therefore, the effective subcarrier set bearing information data is defined as ℳ e = { m | m ∈ [ 1 , M / 2 − 1 ] , m ∈ ℕ } subscript ℳ e conditional-set 𝑚 formulae-sequence 𝑚 1 𝑀 2 1 𝑚 ℕ \mathcal{M}_{\rm e}=\{m|m\in[1,M/2-1],m\in\mathbb{N}\} , where ℕ ℕ \mathbb{N} is the set of natural numbers.
For an ADR, multiple PDs are receiving signals simultaneously. Thus, attention should be paid to the selection of the signal combing schemes. There are different combining schemes such as equal gain combining (EGC), SBC and MRC. An important metric to evaluate the link quality and capacity is the SINR. The SINR of user μ 𝜇 \mu on subcarrier m 𝑚 m can be obtained based on [ 15 ] and [ 27 ] :
When the EGC scheme is adopted, the signals received by the PDs are simply combined with equal weights, which can be described as:
In terms of the SBC scheme, a switch circuit is required to output the information from the PD with the highest SINR. Hence, the weight of each PD is given as:
where p s subscript 𝑝 s p_{\rm{s}} can be obtained by:
On the subject of the MRC schemes, the weight for each PD is denoted as [ 15 ] :
Based on the Shannon capacity, assuming electrical signals after optical to electrical conversion, the data rate of the μ 𝜇 \mu -th UE on subcarrier m 𝑚 m can be expressed as [ 28 ] :

III ADR Structure
The ADR is composed of multiple PDs facing in different directions. By using a PD in conjunction with a compound parabolic concentrator (CPC), a narrow FOV and high optical gain can be achieved [ 11 ] . However, the narrow FOV is achieved at the expense of the longer length of the CPC. Therefore, the number of PDs on the ADR should be limited due to the size limitation on the mobile devices and smartphones. In this study, the TPR [ 21 ] and the PR [ 20 ] are considered as they are both suitable for hand-held devices. The number of PDs on the TPR and PR are separately denoted as N TPR subscript 𝑁 TPR N_{\rm{TPR}} and N PR subscript 𝑁 PR N_{\rm{PR}} . The structure of the TPR with N TPR = 9 subscript 𝑁 TPR 9 N_{\rm{TPR}}=9 and the PR with N PR = 8 subscript 𝑁 PR 8 N_{\rm{PR}}=8 are presented in Fig. 1(b) and 1(a) , respectively. The ADR designs are analyzed in the following parts.
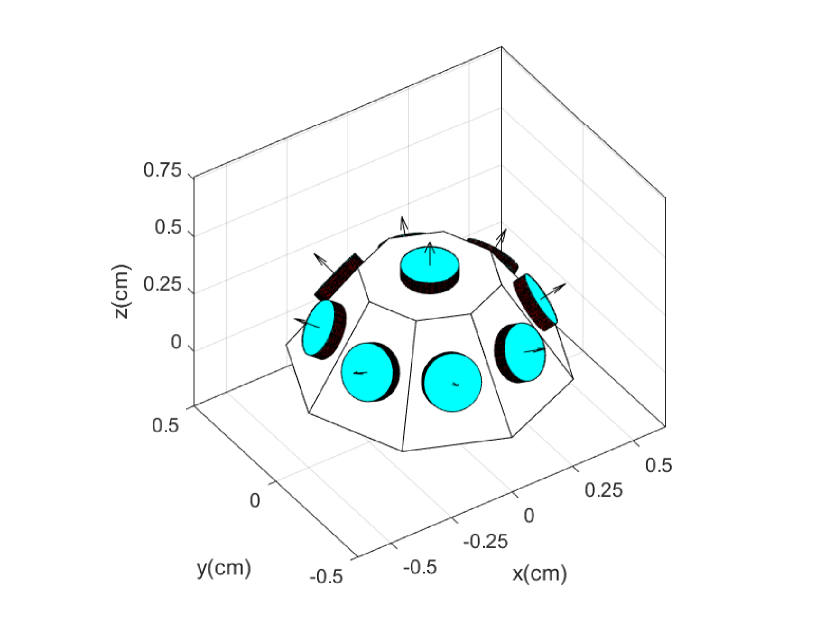
III-A TPR Design
The TPR is composed of a central PD and a ring of N TPR − 1 subscript 𝑁 TPR 1 N_{\rm{TPR}}-1 equally separated side PDs. The side PDs are arranged uniformly in a circle of radius r 𝑟 r on the horizontal plane. Thus, the coordinate of the p 𝑝 p -th PD on a TPR is represented as [ 19 ] :
where ( x UE , y UE , z UE ) subscript 𝑥 UE subscript 𝑦 UE subscript 𝑧 UE (x_{\rm{UE}},y_{\rm{UE}},z_{\rm{UE}}) is the UE position, denoted as 𝐩 UE subscript 𝐩 UE \mathbf{p}_{\rm{UE}} . As the distance between the AP and the UE is much larger than r 𝑟 r , the distances between the AP and all PDs on a TPR are approximately the same. The normal vector of each PD can be described by two angles: the azimuth angle of a PD, ω PD subscript 𝜔 PD \omega_{\rm{PD}} , and the elevation angle of a PD, θ PD subscript 𝜃 PD \theta_{\rm{PD}} [ 18 ] . When the UE is pointing vertically upward, the TPR has one vertically orientated central PD and N TPR − 1 subscript 𝑁 TPR 1 N_{\rm{TPR}}-1 inclined side PDs with identical elevation angles Θ PD subscript Θ PD \Theta_{\rm{PD}} . In other words, the elevation angle of the p 𝑝 p -th PD on a TPR can be expressed as:
The azimuth angle of the p 𝑝 p -th PD is given by:
III-B PR Design
The PR can be regarded as a TPR without the central PD. Therefore, the coordinate of the p 𝑝 p -th PD on a PR is given by:
When the UE is vertically orientated, the elevation angle and the azimuth angle of the p 𝑝 p -th PD are separately expressed as:
III-C Random Orientation Model
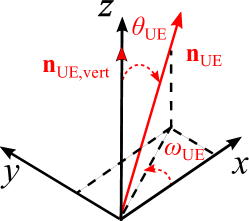
The orientation of a UE has a great impact on the channel DC gain according to ( 2 ). In [ 22 ] , a model for the random orientation of mobile devices based on experiments is proposed so that the system performance of LiFi attocell networks can be evaluated more accurately. The random orientation model can be described by two angles: the elevation angle of a UE, θ UE subscript 𝜃 UE \theta_{\rm{UE}} , and the azimuth angle of a UE, ω UE subscript 𝜔 UE \omega_{\rm{UE}} . The geometrical representation of θ UE subscript 𝜃 UE \theta_{\rm{UE}} and ω UE subscript 𝜔 UE \omega_{\rm{UE}} is manifested in Fig. 2 . The probability density function (PDF) of θ UE subscript 𝜃 UE \theta_{\rm{UE}} can be modeled as the truncated Laplace distribution and it can be simplified as [ 22 ] :
III-D Normal Vector of the ADR
When the UE is pointing vertically upward, for both PRs and TPRs, the normal vector of the p 𝑝 p -th PD is obtained as:
However, the normal vector of the UE will change due to the random rotation. The random orientation model is described in Section III-C . Thus, the normal vector of the p 𝑝 p -th PD after the random rotation is obtained by:
and the azimuth angle of the p 𝑝 p -th PD can be expressed as:
III-E Receiver Bandwidth vs PD Area
The bandwidth of a PD is affected by its physical area, A p subscript 𝐴 p A_{\rm{p}} , and the PD thickness, L p subscript 𝐿 p L_{\rm{p}} . The capacitance of the each PD is denoted as C r = ε 0 ε r A p L p subscript 𝐶 r subscript 𝜀 0 subscript 𝜀 r subscript 𝐴 p subscript 𝐿 p C_{\rm{r}}=\varepsilon_{0}\varepsilon_{\rm{r}}\frac{A_{\rm{p}}}{L_{\rm{p}}} , where ε 0 subscript 𝜀 0 \varepsilon_{0} and ε r subscript 𝜀 r \varepsilon_{\rm{r}} are the permittivity of vacuum and and the relative permittivity of silicon, respectively. The load resistance is defined as R load subscript 𝑅 load R_{\rm{load}} while the hole velocity is denoted as v p subscript 𝑣 p v_{\rm{p}} . Therefore, the receiver bandwidth can be written as [ 30 ] :
III-F Visibility of an ADR
where 𝐝 α subscript 𝐝 𝛼 \mathbf{d}_{\alpha} = ( x α − x UE , y α − y UE , z α − z UE ) subscript 𝑥 𝛼 subscript 𝑥 UE subscript 𝑦 𝛼 subscript 𝑦 UE subscript 𝑧 𝛼 subscript 𝑧 UE (x_{\alpha}-x_{\rm{UE}},y_{\alpha}-y_{\rm{UE}},z_{\alpha}-z_{\rm{UE}}) is the distance vector between the AP α 𝛼 \alpha and the UE. The dot product is denoted as ( ⋅ ) ⋅ (\cdot) and ∥ ⋅ ∥ delimited-∥∥ ⋅ \left\lVert\cdot\right\rVert is the norm operator. In terms of ADR, an AP is visible to an ADR if and only if the AP is visible to at least one of the PDs on the ADR. Hence, for a given UE position and orientation, the visibility of the ADR can be written as [ 18 ] :
It is assumed that both x UE subscript 𝑥 UE x_{\rm{UE}} and y UE subscript 𝑦 UE y_{\rm{UE}} follow a uniform distribution. The probability of visibility of an ADR is defined as the probability that there is at least one AP within the visible area of the ADR for all UE positions and orientations, and it can be expressed as follows:
where X UE subscript 𝑋 UE X_{\rm{UE}} , Y UE subscript 𝑌 UE Y_{\rm{UE}} and Ω UE subscript Ω UE \Omega_{\rm{UE}} are the range of x UE subscript 𝑥 UE x_{\rm{UE}} , y UE subscript 𝑦 UE y_{\rm{UE}} and ω UE subscript 𝜔 UE \omega_{\rm{UE}} , respectively. Hence, it can be obtained that X UE = max ( x UE ) − min ( x UE ) subscript 𝑋 UE subscript 𝑥 UE subscript 𝑥 UE X_{\rm{UE}}=\max(x_{\rm{UE}})-\min(x_{\rm{UE}}) , Y UE = max ( y UE ) − min ( y UE ) subscript 𝑌 UE subscript 𝑦 UE subscript 𝑦 UE Y_{\rm{UE}}=\max(y_{\rm{UE}})-\min(y_{\rm{UE}}) and Ω UE = max ( ω UE ) − min ( ω UE ) subscript Ω UE subscript 𝜔 UE subscript 𝜔 UE \Omega_{\rm{UE}}=\max(\omega_{\rm{UE}})-\min(\omega_{\rm{UE}}) .
IV The Optimum Field of View
Iv-a optimization problem.
In ( 2 ), the LOS channel gain H LOS subscript 𝐻 LOS H_{\rm{LOS}} is a convex function of Ψ c subscript Ψ c \Psi_{\rm{c}} and decreases monotonically. Hence, the smaller the Ψ c subscript Ψ c \Psi_{\rm{c}} , the higher the channel gain. However, when the Ψ c subscript Ψ c \Psi_{\rm{c}} of the PD is too small, there is a high chance that no APs are visible to the ADR and the LOS link cannot be constructed. Thus, there is a trade off between the LOS channel gain and visibility. The optimization problem is formulated as maximizing the LOS channel gain based on the constraint that the ADR should provide visibility for all UE locations. Thus, the optimization function is written as:
IV-B Coverage Area of ADR on the Ceiling
The coverage area of a PR for a vertical-orientated UE is studied in [ 18 ] .
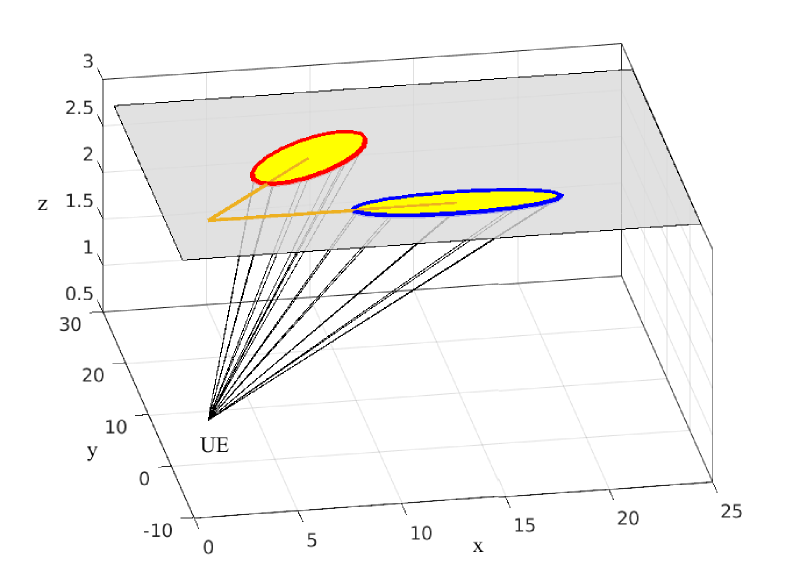
The TPR can be seen as the combination of a PR, where N PR = N TPR − 1 subscript 𝑁 PR subscript 𝑁 TPR 1 N_{\rm{PR}}=N_{\rm{TPR}}-1 , and a central PD. When the UE is facing vertically upward, the visible area of the central PD is a circle. Therefore, the shape of the visible area of the p 𝑝 p -th PD on a TPR is given by:
superscript subscript 𝑥 circle 2 superscript subscript 𝑦 circle 2 superscript ℎ subscript Ψ c 2 x_{\rm{circle}}^{2}+y_{\rm{circle}}^{2}=(h\tan\Psi_{\rm{c}})^{2} .
IV-C Lower Bound of FOV
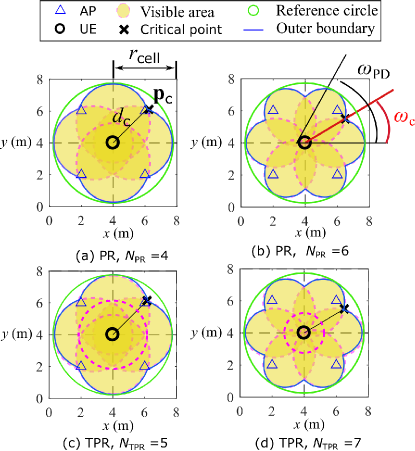
For a fixed UE location, the ADR has the smallest coverage area on the ceiling when vertically orientated. In other words, we will investigate the worst condition, i.e. the situation that an ADR is positioned vertically upward which provides the smallest coverage area on the ceiling. Under other orientation scenarios, the coverage area is larger. Based on ( 30 ) and ( 31 ), Fig. 4 illustrates the visible area of 4 different types of ADRs when the UE is at the cell corner, that is to say, the cross-point of four LiFi cells. The blue curve is the outer boundary of the visible area. On the outer boundary, the points that have the shortest distance to the UE are defined as critical points, 𝐩 c subscript 𝐩 c \mathbf{p}_{\rm{c}} . d c subscript 𝑑 c d_{\rm{c}} denotes the horizontal distance between 𝐩 c subscript 𝐩 c \mathbf{p}_{\rm{c}} and the UE. To ensure p v = 1 subscript 𝑝 v 1 p_{\rm{v}}=1 , there are two constraints and the detailed explanation of these constraints are given as follows:
IV-C 1 Constraint \@slowromancap i @ \@slowromancap 𝑖 @ \@slowromancap i@
The central area above the ADR should be visible to the ADR.
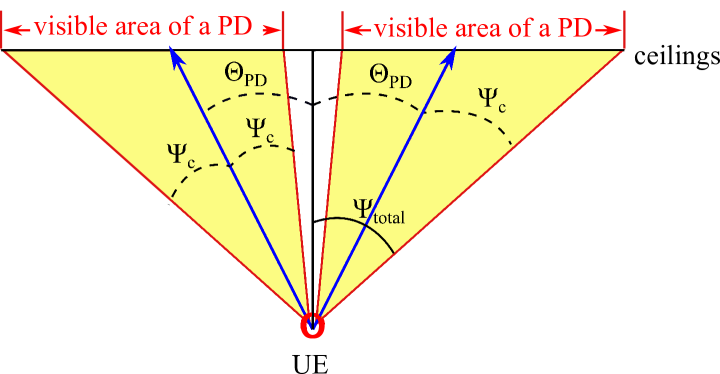
As shown in Fig. 5(a) , the total FOV of an ADR is represented as Ψ total subscript Ψ total \Psi_{\rm{total}} , which can be written as:
In terms of PRs, if Θ PD ≥ Ψ c subscript Θ PD subscript Ψ c \Theta_{\rm{PD}}\geq\Psi_{\rm{c}} , then the central part is not covered by the visible area of the ADR as manifested in Fig. 5(a) . If the UE is in the cell center, then no APs will be visible to the ADR. Hence, the condition Θ PD ≤ Ψ c subscript Θ PD subscript Ψ c \Theta_{\rm{PD}}\leq\Psi_{\rm{c}} should be satisfied so that the area directly above the UE is covered by the visible area of the ADR. Based on this constraint and ( 32 ), the lower bound of Ψ c subscript Ψ c \Psi_{\rm{c}} can be obtained as:
With respect to the TPR, the area directly above the UE is covered by the central PD orientating vertically upwards as illustrated in Fig. 5(b) . The concern should be the central coverage gap between the central PD and the side PDs. Therefore, Θ PD ≤ 2 Ψ c subscript Θ PD 2 subscript Ψ c \Theta_{\rm{PD}}\leq 2\Psi_{\rm{c}} is required to ensure there is no gap between them. By substituting this constraint into ( 32 ), it can be derived that:
IV-C 2 Constraint \@slowromancap i i @ \@slowromancap 𝑖 𝑖 @ \@slowromancap ii@
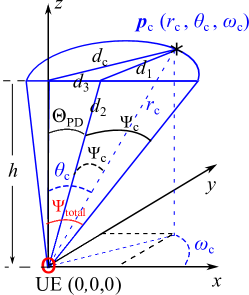
According to ( 37 ), ( 36 ) and ( 38 ), the lower bound of Ψ c subscript Ψ c \Psi_{\rm{c}} set by Constraint \@slowromancap ii @ \@slowromancap ii @ \rm{\@slowromancap ii@} is derived in Appendix B and is represented as:
IV-C 3 Summary
Based on ( 33 ), ( 34 ) and ( 39 ), the lower bound of Ψ c subscript Ψ c \Psi_{\rm{c}} can be expressed as:
V Double Source Cell Configuration
In the conventional SS cell configuration, each cell is equipped with a single AP in the cell center. The double source (DS) cell configuration is proposed to further exploit the spatial diversity of the ADR in [ 17 ] . As demonstrated in Fig. 7 , each LiFi AP consists of two sources which transmit the same information signals but with opposite polarity. These two sources are termed as the positive source and the negative source, which transmit the time domain signal s pos ( t ) subscript 𝑠 pos 𝑡 s_{\rm{pos}}(t) and s neg ( t ) subscript 𝑠 neg 𝑡 s_{\rm{neg}}(t) respectively. In a single optical cell, the received optical signal at a PD is denoted as [ 17 ] :
where H pos subscript 𝐻 pos H_{\rm{pos}} is the channel gain between the positive source and the PD; H neg subscript 𝐻 neg H_{\rm{neg}} is the channel gain between the negative source and the PD. For a fair comparison, the total transmitting power for the SS system and DS system should be the same. Hence, the transmit power of each source is halved when the DS configuration is applied and the received optical power at the PD can be written as [ 17 ] :
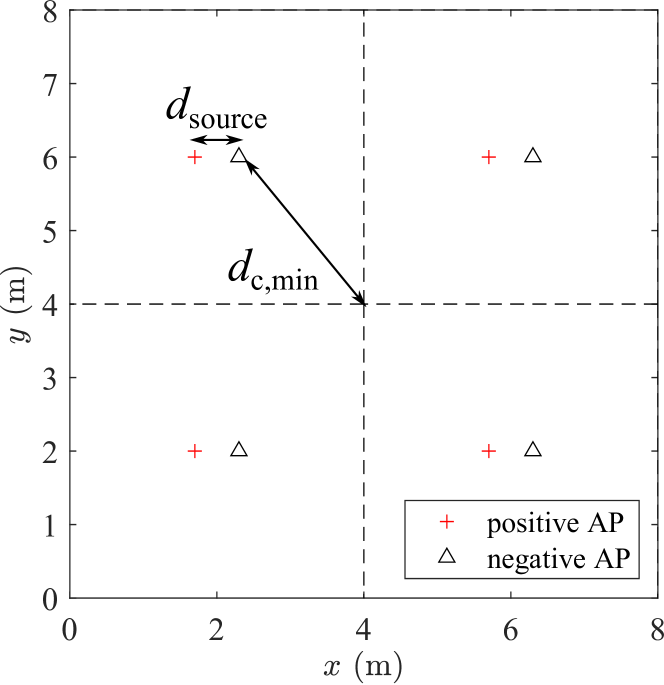
Generally, the receiver is closer to the desired AP than the interfering AP. For the desired AP, due to the narrow FOV of ADRs, one PD can hardly receive LOS signals from both the positive source and negative source simultaneously, and only one appears as the LOS channel gain. In respect of the interfering AP, the channel gains H pos subscript 𝐻 pos H_{\rm{pos}} and H neg subscript 𝐻 neg H_{\rm{neg}} are both NLOS. Hence, the difference between H pos subscript 𝐻 pos H_{\rm{pos}} and H neg subscript 𝐻 neg H_{\rm{neg}} is small and the interference is attenuated accordingly. Therefore, the double source cell configuration can suppress the signal power from interfering APs [ 17 ] . As the LOS interference can be mitigated by the narrow FOV of the ADR and the NLOS interference can be mitigated due to the adoption of the DS configuration, the SINR of user μ 𝜇 \mu on subcarrier m 𝑚 m can be approximated by:
Therefore, the lower bound of Ψ c subscript Ψ c \Psi_{\rm{c}} for the double source cell system can be calculated based on ( 39 ) - ( 42 ).
VI Results and Discussions
Vi-a simulation setups.
As shown in Fig. 4 , an 8 m × \times 8 m × \times 3 m indoor office scenario is considered in this study, where 4 LiFi APs are deployed following a square topology. All of the users are uniformly distributed in the room and move randomly following the random waypoint model [ 22 ] . To make a fair comparison, the total physical area, A t = N PD A p subscript 𝐴 t subscript 𝑁 PD subscript 𝐴 p A_{\rm{t}}=N_{\rm{PD}}A_{\rm{p}} , of the ADRs should be the same. Hence, the physical area A p subscript 𝐴 p A_{\rm{p}} on each PD decreases when the number of PDs increases. The other parameters used in the simulations are listed in Table I .
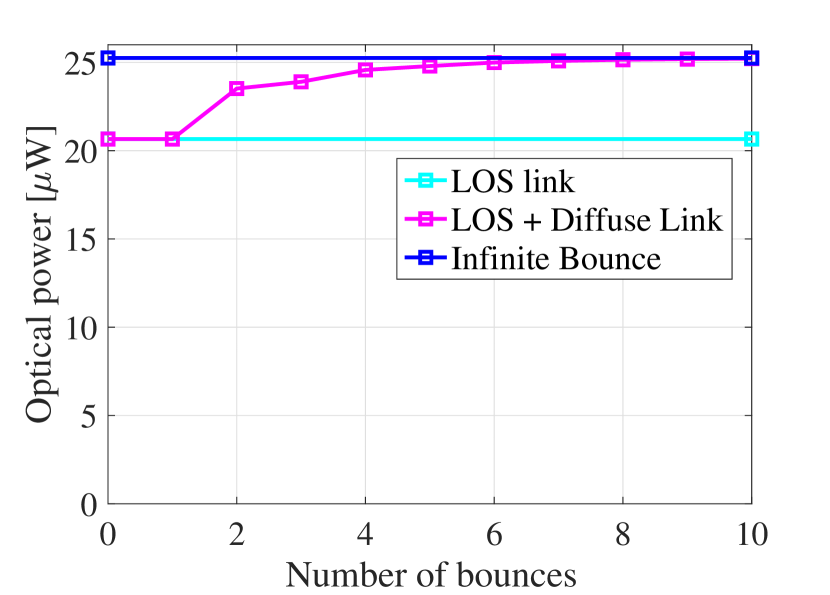
VI-B Importance of Reflection and Orientation
Fig. 8 shows the received optical power in two different locations with different orientations. The room setup and the SS deployment is as shown in Fig. 5 and a single PD receiver with a FOV of 60 ∘ superscript 60 60^{\circ} is used. The received optical power from the LOS and NLOS link can be calculated based on ( 2 ) and ( 3 ), respectively. The ratio of the received optical power from the LOS signal link to the total received optical power is represented as p los subscript 𝑝 los p_{\rm{los}} . In Fig. 8(a) and 8(b) , the p los subscript 𝑝 los p_{\rm{los}} of cell-center UEs at (6,6) degrades substantially when the orientation changes. In Fig. 8(c) , the optical power from the diffuse link occupies more than 40 % percent 40 40\% of the total optical power when x UE = 1 , y UE = 1 formulae-sequence subscript 𝑥 UE 1 subscript 𝑦 UE 1 x_{\rm{UE}}=1,y_{\rm{UE}}=1 . Nevertheless, in Fig. 8(d) , by changing the device orientation, there is no LOS signal and only the signal from the diffuse links can be received. Therefore, the device orientation has a great impact on the received single power and thus cannot be ignored. In addition, both the LOS link and diffuse link should be considered to analyze the performance of a multi-cell visible light communication system. Fig. 8 demonstrates that when the number of bounces is more than 5, the corresponding paths make a minor contribution to the total optical power. Hence, to reduce the computational complexity while maintaining high channel estimation accuracy, a light reflection order of L = 5 𝐿 5 L=5 and the orientation model proposed in [ 22 ] are considered for the following simulations.
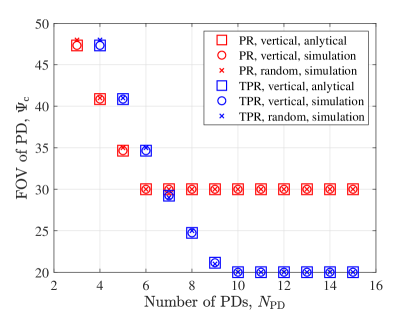
VI-C Performance Analysis for SS cells
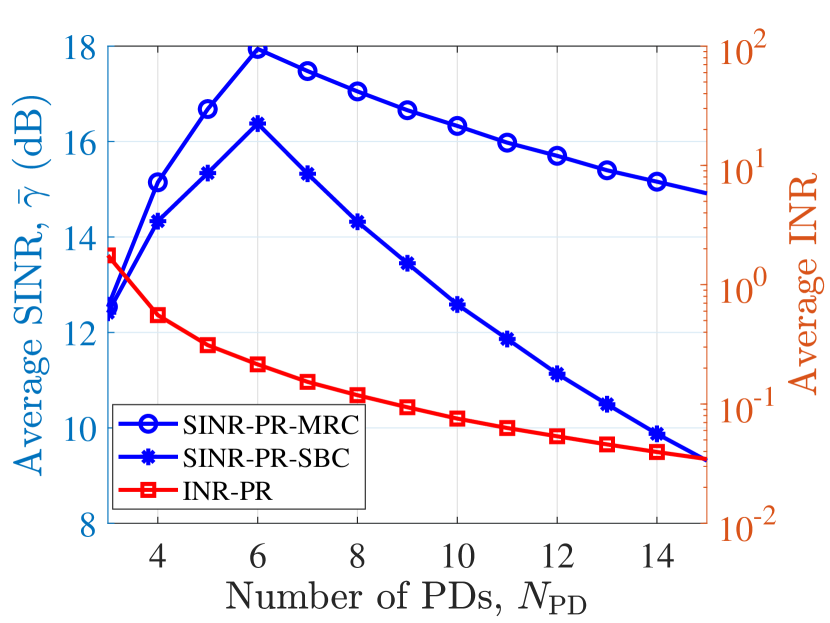
2) MRC vs. SBC : The MRC scheme is known to achieve better performance than the SBC scheme when there is no interference in the system. However, this may not be true when the interference is taken into consideration. To demonstrate the performance comparison between the MRC and SBC scheme, the simulation is carried out for different noise levels. In Fig. 10(a) , the noise power spectrum density level N 0 subscript 𝑁 0 N_{0} is 10 − 20 superscript 10 20 10^{-20} A 2 /Hz. When N PD = 3 subscript 𝑁 PD 3 N_{\rm{PD}}=3 , the level of interference is slightly higher than the noise, and the MRC schemes achieves similar average SINR as the SBC scheme. With the increase of N PD subscript 𝑁 PD N_{\rm{PD}} , the noise level becomes higher than the interference level and the system gradually becomes noise dominated. It can be seen that in a noise-limited system, MRC outperforms SBC in terms of average SINR. The noise power spectrum density level N 0 subscript 𝑁 0 N_{0} is 10 − 21 superscript 10 21 10^{-21} A 2 /Hz in Fig. 10(b) . When N PD ≤ 6 subscript 𝑁 PD 6 N_{\rm{PD}}\leq 6 , the interference level is higher than the noise level and SBC performs slightly better than MRC. However, the noise starts to rise above the interference level with the further increase of N PD subscript 𝑁 PD N_{\rm{PD}} and thus MRC outperforms SBC. For a noise power spectrum density level of 10 − 22 superscript 10 22 10^{-22} A 2 /Hz, Fig. 10(c) depicts an interference-limited system and the SBC scheme achieves a higher average SINR than the MRC scheme for all values of N PD subscript 𝑁 PD N_{\rm{PD}} . In brief, when the system is interference dominated, SBC is a better combining scheme. Otherwise, MRC outperforms SBC when considering the average SINR.
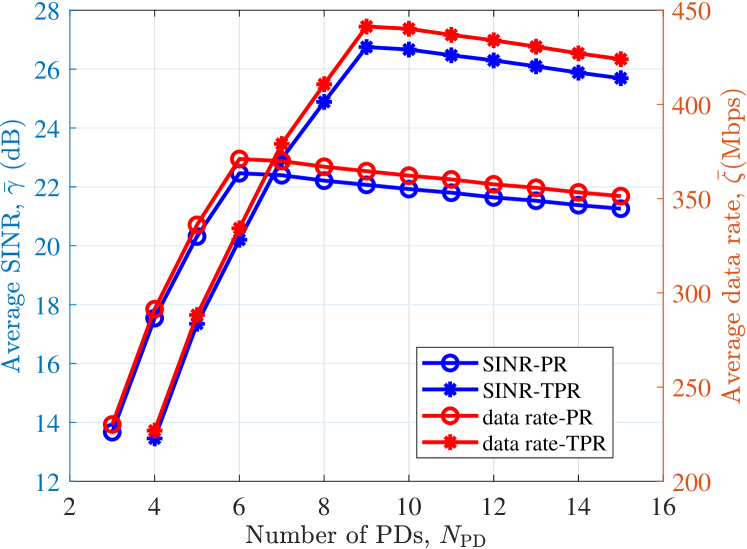
4) Receiver bandwidth vs. transmitter bandwidth : Based on ( 22 ), the relationship between the receiver bandwidth, B r subscript 𝐵 r B_{\rm{r}} , and the number of PDs, N PD subscript 𝑁 PD N_{\rm{PD}} , is manifested in Fig. 12 . When N PD subscript 𝑁 PD N_{\rm{PD}} increases from 3 to 15, the bandwidth increases from 150 MHz to around 350 MHz due to the decrease in the physical area of each PD. The communication bandwidth B L subscript 𝐵 L B_{\rm{L}} is the minimum value between the receiver bandwidth and the transmitter bandwidth, which is denoted as B L = min ( B r , B t ) subscript 𝐵 L subscript 𝐵 r subscript 𝐵 t B_{\rm{L}}=\min(B_{\rm{r}},B_{\rm{t}}) . The user data rate is determined by the SINR and the communication bandwidth. When the transmitter bandwidth B t subscript 𝐵 t B_{\rm{t}} is 100 MHz, which is less than B r subscript 𝐵 r B_{\rm{r}} for all values of N PD subscript 𝑁 PD N_{\rm{PD}} in Fig. 12 , the communication bandwidth is limited by the transmitter and thus B L = 100 subscript 𝐵 L 100 B_{\rm{L}}=100 MHz. As B L subscript 𝐵 L B_{\rm{L}} does not vary according to N PD subscript 𝑁 PD N_{\rm{PD}} , the average data rate follows the same trend as the average SINR in Fig. 11(a) . By increasing the transmitter bandwidth B t subscript 𝐵 t B_{\rm{t}} to 500 MHz, B r ≤ B t subscript 𝐵 r subscript 𝐵 t B_{\rm{r}}\leq B_{\rm{t}} for all N PD subscript 𝑁 PD N_{\rm{PD}} and the communication bandwidth is limited by the receiver side. Hence, the communication bandwidth B L = B r subscript 𝐵 L subscript 𝐵 r B_{\rm{L}}=B_{\rm{r}} . In Fig. 11(b) , with the increase of N PD subscript 𝑁 PD N_{\rm{PD}} , the average SINR first increases and then decreases, which peaks at N PD = 6 subscript 𝑁 PD 6 N_{\rm{PD}}=6 and N PD = 9 subscript 𝑁 PD 9 N_{\rm{PD}}=9 for the PR and TPR, respectively. In contrast, when N PD subscript 𝑁 PD N_{\rm{PD}} grows, the average data rate increases even when the SINR degrades, which is due to the greater bandwidth. To sum up, the PR with N PD = 6 subscript 𝑁 PD 6 N_{\rm{PD}}=6 and TPR with N PD = 9 subscript 𝑁 PD 9 N_{\rm{PD}}=9 achieve the highest average data rate for transmitter-bandwidth-limited systems whereas the average data rate peaks at N PD = 15 subscript 𝑁 PD 15 N_{\rm{PD}}=15 in a receiver-bandwidth-limited system for both PR and TPR.
VI-D Performance Analysis for DS cells
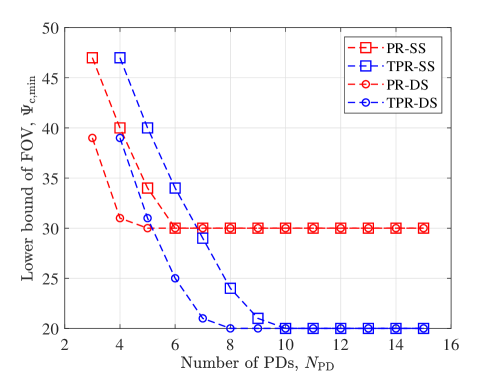
2) MRC vs. SBC : Previously, in Fig. 10(c) , we have shown the performance comparison between the MRC and SBC for the SS system with N 0 = 10 − 22 subscript 𝑁 0 superscript 10 22 N_{0}=10^{-22} A 2 /Hz. Due to the low level of noise power spectral density, the system is mostly interference-limited and SBC outperforms MRC for all given values of N PD subscript 𝑁 PD N_{\rm{PD}} . By adopting the DS configuration, as shown in Fig. 14 , the average interference to noise ratio (INR) is larger than 1 only when there are 3 or 4 PDs on the PR. Whereas for N PD ≥ 5 subscript 𝑁 PD 5 N_{\rm{PD}}\geq 5 , the noise plays a similar or more important role than the interference. Therefore, compared with the SS system, for the same level of noise power spectral density, the average INR of the DS system degrades substantially. This indicates that the DS system can mitigate interference. With regards to the average SINR, MRC and SBC have similar performance when N PD ≤ 4 subscript 𝑁 PD 4 N_{\rm{PD}}\leq 4 whereas MRC outperforms SBC for N PD > 4 subscript 𝑁 PD 4 N_{\rm{PD}}>4 . From the above analyses, it can be deducted that MRC is a better combining scheme for the DS system with respect to the three different levels of N 0 subscript 𝑁 0 N_{0} given previously in this study.
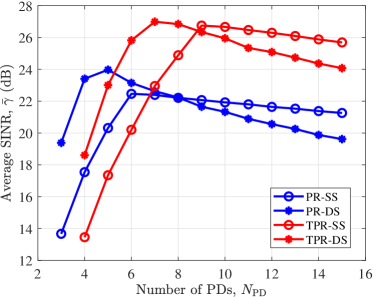
4) DS vs. SS : Fig. 16 demonstrates the performance comparison between the DS system and the SS system with respect to different levels of noise power spectrum density, N 0 subscript 𝑁 0 N_{0} . The typical value of N 0 subscript 𝑁 0 N_{0} for a PD is 10 − 21 superscript 10 21 10^{-21} A 2 /Hz, at which the DS system achieves a slightly higher average SINR than the SS system for both PRs and TPRs. By reducing the noise power spectrum density level to 10 − 22 superscript 10 22 10^{-22} A 2 /Hz, the system becomes interference-limited. With the aid of the DS configuration, the ADR can suppress the signal power from interfering APs by attenuating the NLOS path. Hence, when N 0 = 10 − 22 subscript 𝑁 0 superscript 10 22 N_{0}=10^{-22} A 2 /Hz, the average SINR of DS cells is 7 dB and 5 dB higher than the average SINR of SS cells for PRs and TPRs, respectively. For a noise spectrum density level of 10 − 20 superscript 10 20 10^{-20} A 2 /Hz, the system becomes noise limited, in which the DS could not improve system performance by reducing the power of interference signal. In addition, the received power is halved when the DS configuration is applied, and thus the SS outperforms the DS in terms of the average SINR.
VII Conclusions
This paper investigates the ICI mitigation in LiFi networks using ADRs. The impact of the diffuse link considering random UE orientation is studied and it is shown that both LOS and diffuse links have an important influence on the system performance. The performance of different ADR structures are compared and the optimized ADR structure is proposed for the considered scenario, where the method can be extended to other scenarios easily. By studying systems with different levels of noise power spectrum density, we showed that when the system is noise-limited, MRC outperforms SBC, otherwise, SBC is the preferred combining scheme. In an interference-limited system or noise-plus-interference limited system, the adoption of the DS cell configuration can further mitigate the NLOS interference and thus improve the system performance. However, the limitation of the DS cell is that the transmit power is equally split to the positive and negative sources, which degrades the performance of the noise-limited system.
Appendix A Proof of ( 27 )
The visible area of the 1-st PD with ω PD 1 = 0 subscript superscript 𝜔 1 PD 0 \omega^{1}_{\rm{PD}}=0 is illustrated in Fig. 6 a. The point O represents the location of the UE, 𝐩 UE subscript 𝐩 UE \mathbf{p}_{\rm{UE}} , and 𝐎𝐄 𝐎𝐄 \mathbf{OE} represents the normal vector of the PD. The intersection point of the normal vector with the ceiling is 𝐄 𝐄 \mathbf{E} . The coordinate of 𝐄 𝐄 \mathbf{E} is denoted as ( x e , y e , z e ) subscript 𝑥 e subscript 𝑦 e subscript 𝑧 e (x_{\rm{e}},y_{\rm{e}},z_{\rm{e}}) and points 𝐀 𝐀 \mathbf{A} , 𝐁 𝐁 \mathbf{B} , 𝐂 𝐂 \mathbf{C} , 𝐃 𝐃 \mathbf{D} , 𝐅 𝐅 \mathbf{F} are denoted in the same way. Points 𝐀 𝐀 \mathbf{A} and 𝐁 𝐁 \mathbf{B} are the vertices of the ellipse. Points 𝐂 𝐂 \mathbf{C} and 𝐃 𝐃 \mathbf{D} are the co-vertices of the ellipse. The centre point of the ellipse is denoted as 𝐅 𝐅 \mathbf{F} . The angle between each of the four vectors, 𝐎𝐀 𝐎𝐀 \mathbf{OA} , 𝐎𝐁 𝐎𝐁 \mathbf{OB} , 𝐎𝐂 𝐎𝐂 \mathbf{OC} , 𝐎𝐃 𝐎𝐃 \mathbf{OD} with 𝐎𝐄 𝐎𝐄 \mathbf{OE} is Ψ c subscript Ψ c \Psi_{\rm{c}} . The length of the semi-major and semi-minor axes of the ellipse are represented by a 𝑎 a and b 𝑏 b separately. The length of semi-major axes a 𝑎 a is denoted as:
As 𝐀 𝐀 \mathbf{A} , 𝐁 𝐁 \mathbf{B} , 𝐄 𝐄 \mathbf{E} , 𝐅 𝐅 \mathbf{F} and 𝐎 𝐎 \mathbf{O} are on the same x z 𝑥 𝑧 xz -plane, y a = y b = y e = y f = y UE subscript 𝑦 a subscript 𝑦 b subscript 𝑦 e subscript 𝑦 f subscript 𝑦 UE y_{\rm{a}}=y_{\rm{b}}=y_{\rm{e}}=y_{\rm{f}}=y_{\rm{UE}} . As 𝐀 𝐀 \mathbf{A} , 𝐁 𝐁 \mathbf{B} , 𝐂 𝐂 \mathbf{C} , 𝐃 𝐃 \mathbf{D} , 𝐄 𝐄 \mathbf{E} and 𝐅 𝐅 \mathbf{F} are on the ceiling, z a = z b = z c = z d = z f = z e = z AP subscript 𝑧 a subscript 𝑧 b subscript 𝑧 c subscript 𝑧 d subscript 𝑧 f subscript 𝑧 e subscript 𝑧 AP z_{\rm{a}}=z_{\rm{b}}=z_{\rm{c}}=z_{\rm{d}}=z_{\rm{f}}=z_{\rm{e}}=z_{\rm{AP}} , where z AP subscript 𝑧 AP z_{\rm{AP}} is the height of the AP. As 𝐂 𝐂 \mathbf{C} and 𝐃 𝐃 \mathbf{D} are on the same y z 𝑦 𝑧 yz -plane with 𝐅 𝐅 \mathbf{F} , the coordinates x 𝑥 x of these points are represented by:
2 subscript Ψ c 2 subscript Θ PD 𝑏 ℎ \mathbf{OC}=(\frac{h\sin(2\Theta_{\rm{PD}})}{\cos(2\Psi_{\rm{c}})+\cos(2\Theta_{\rm{PD}})},b,h) . Since cos ( Ψ c ) = 𝐎𝐄 ⋅ 𝐎𝐂 | 𝐎𝐄 | | 𝐎𝐂 | subscript Ψ c ⋅ 𝐎𝐄 𝐎𝐂 𝐎𝐄 𝐎𝐂 \cos(\Psi_{\rm{c}})=\frac{\mathbf{OE}\cdot\mathbf{OC}}{|\mathbf{OE}||\mathbf{OC}|} , b 𝑏 b can be obtained as:
Consequently, the equation of the ellipse is thus given by:
Appendix B Proof of ( 39 )
Substituting ( 37 ) and ( 38 ) into ( 36 ), we can get:
Substituting ( 32 ) into ( 51 ), the elevation angle of each PD, Θ PD subscript Θ PD \Theta_{\rm{PD}} , on PRs is derived as:
The function F 1 subscript 𝐹 1 F_{1} has one zero at z 1 = h tan ( Ψ total ) subscript 𝑧 1 ℎ subscript Ψ total z_{1}=h\tan(\Psi_{\rm{total}}) and one pole at p 1 = h sin ( Ψ total ) cos 2 ( Ψ total ) − sin 2 ( ω c ) subscript 𝑝 1 ℎ subscript Ψ total superscript 2 subscript Ψ total superscript 2 subscript 𝜔 c p_{1}=\frac{h\sin(\Psi_{\rm{total}})}{\sqrt{\cos^{2}(\Psi_{\rm{total}})-\sin^{2}(\omega_{\rm{c}})}} . The derivative of F 1 ( d c ) subscript 𝐹 1 subscript 𝑑 c F_{1}(d_{\rm{c}}) is given by:
subscript 𝑑 c 0 \frac{\partial F_{1}}{\partial d_{\rm{c}}}=0 , the two roots are denoted as:
2 subscript 𝑑 c 0 \frac{\partial^{2}F_{1}(d_{\rm{c2}})}{\partial^{2}d_{\rm{c}}}<0 . Similarly, for d c ∈ ( 0 , h tan ( Ψ total ) ] subscript 𝑑 c 0 ℎ subscript Ψ total d_{\rm{c}}\in(0,h\tan(\Psi_{\rm{total}})] , there is a local maximum at d c2 subscript 𝑑 c2 d_{\rm{c2}} . In summary, the upper bound of F 1 subscript 𝐹 1 F_{1} is thus given by:
According to ( 32 ), Ψ c subscript Ψ c \Psi_{\rm{c}} is given by:
Hence, the lower bound of F 2 subscript 𝐹 2 F_{2} is denoted as:
- [1] D. C. O’Brien, “Visible Light Communications: Challenges and potential.” IEEE, October 2011, pp. 365–366.
- [2] H. Haas, L. Yin, Y. Wang, and C. Chen, “What is LiFi?” Journal of Lightwave Technology , vol. 34, no. 6, pp. 1533–1544, March 2016.
- [3] S. Wu, H. Wang, and C. H. Youn, “Visible Light Communications for 5G Wireless Networking Systems: from Fixed to Mobile Communications,” IEEE Network , vol. 28, no. 6, pp. 41–45, Nov 2014.
- [4] M. S. Alouini and A. J. Goldsmith, “Area Spectral Efficiency of Cellular Mobile Radio Systems,” Vehicular Technology, IEEE Transactions on , vol. 48, no. 4, pp. 1047–1066, 1999.
- [5] T. Borogovac, M. Rahaim, and J. B. Carruthers, “Spotlighting for Visible Light Communications and Illumination,” in 2010 IEEE Globecom Workshops , Dec 2010, pp. 1077–1081.
- [6] G. W. Marsh and J. M. Kahn, “Channel Reuse Strategies for Indoor Infrared Wireless Communications,” Communications, IEEE Transactions on , vol. 45, no. 10, pp. 1280–1290, 1997.
- [7] K. Cui, J. Quan, and Z. Xu, “Performance of Indoor Optical Femtocell by Visible Light Communication,” Optics Communications , vol. 298-299, pp. 59–66, 2013.
- [8] C. Chen, S. Videv, D. Tsonev, and H. Haas, “Fractional Frequency Reuse in DCO- OFDM- Based Optical Attocell Networks,” Lightwave Technology, Journal of , vol. 33, no. 19, pp. 3986–4000, 2015.
- [9] C. Chen, D. Tsonev, and H. Haas, “Joint Transmission in Indoor Visible Light Communication Downlink Cellular Networks,” in 2013 IEEE Globecom Workshops (GC Wkshps) , Dec 2013, pp. 1127–1132.
- [10] Z. Chen, D. A. Basnayaka, and H. Haas, “Space Division Multiple Access for Optical Attocell Network Using Angle Diversity Transmitters,” Journal of Lightwave Technology , vol. 35, no. 11, pp. 2118–2131, June 2017.
- [11] J. M. Kahn and J. R. Barry, “Wireless Infrared Communications,” Proceedings of the IEEE , vol. 85, no. 2, pp. 265–298, 1997.
- [12] J. Carruthers and J. Kahn, “Angle diversity for nondirected wireless infrared communication,” in ICC ’98. 1998 IEEE International Conference on Communications. Conference Record. Affiliated with SUPERCOMM’98 (Cat. No.98CH36220) , vol. 3, 1998, pp. 1665–1670 vol.3.
- [13] J. Carruther and J. Kahn, “Angle diversity for nondirected wireless infrared communication,” IEEE Transactions on Communications , vol. 48, no. 6, pp. 960–969, 2000.
- [14] G. Yun and M. Kavehrad, “Spot-diffusing and fly-eye receivers for indoor infrared wireless communications,” in 1992 IEEE International Conference on Selected Topics in Wireless Communications , 1992, pp. 262–265.
- [15] Z. Chen, D. Basnayaka, X. Wu, and H. Haas, “Interference Mitigation for Indoor Optical Attocell Networks using Angle Diversity Receiver,” Journal of Lightwave Technology , pp. 1–1, 2018.
- [16] Z. Chen, N. Serafimovski, and H. Haas, “Angle Diversity for an Indoor Cellular Visible Light Communication System,” in 2014 IEEE 79th Vehicular Technology Conference (VTC Spring) , May 2014, pp. 1–5.
- [17] Z. Chen, D. Tsonev, and H. Haas, “A Novel Double-Source Cell Configuration for Indoor Optical Attocell Networks,” in 2014 IEEE Global Communications Conference , Dec 2014, pp. 2125–2130.
- [18] Z. Zeng, M. D. Soltani, M. Safari, and H. Haas, “Angle Diversity Receiver in LiFi Cellular Networks,” in ICC 2019 - 2019 IEEE International Conference on Communications (ICC) , May 2019, pp. 1–6.
- [19] Z. Zeng, M. D. Soltani, X. Wu, and H. Haas, “Access Point Selection Scheme for LiFi Cellular Networks using Angle Diversity Receivers,” in 2019 IEEE Wireless Communications and Networking Conference (WCNC) , 2019, pp. 1–6.
- [20] A. Nuwanpriya, S. W. Ho, and C. S. Chen, “Indoor MIMO Visible Light Communications: Novel Angle Diversity Receivers for Mobile Users,” IEEE Journal on Selected Areas in Communications , vol. 33, no. 9, pp. 1780–1792, Sept 2015.
- [21] C. Chen, W. D. Zhong, H. Yang, S. Zhang, and P. Du, “Reduction of SINR Fluctuation in Indoor Multi-Cell VLC Systems Using Optimized Angle Diversity Receiver,” Journal of Lightwave Technology , pp. 1–1, 2018.
- [22] M. D. Soltani, A. A. Purwita, Z. Zeng, H. Haas, and M. Safari, “Modeling the Random Orientation of Mobile Devices: Measurement, Analysis and LiFi Use Case,” IEEE Transactions on Communications , vol. 67, no. 3, pp. 2157–2172, March 2019.
- [23] Z. Zeng, M. D. Soltani, H. Haas, and M. Safari, “Orientation Model of Mobile Device for Indoor VLC and Millimetre Wave Systems,” Accepted in 2018 IEEE 88nd Vehicular Technology Conference (VTC2018-Fall) , Chicago, USA, August 2018.
- [24] C. Chen, D. A. Basnayaka, and H. Haas, “Downlink Performance of Optical Attocell Networks,” Journal of Lightwave Technology , vol. 34, no. 1, pp. 137–156, Jan 2016.
- [25] H. Schulze, “Frequency-Domain Simulation of the Indoor Wireless Optical Communication Channel,” IEEE Transactions on Communications , vol. 64, no. 6, pp. 2551–2562, June 2016.
- [26] C. Chen, D. Basnayaka, and H. Haas, “Downlink Performance of Optical Attocell Networks,” Journal of Lightwave Technology , vol. 34, no. 1, pp. 137–156, Apr. 2016.
- [27] C. Chen et al. , “Downlink Performance of Optical Attocell Networks,” Journal of Lightwave Technology , vol. 34, no. 1, pp. 137–156, Jan 2016.
- [28] S. Dimitrov and H. Haas, “Information Rate of OFDM-Based Optical Wireless Communication Systems With Nonlinear Distortion,” J. Lightwave Technol. , vol. 31, no. 6, pp. 918–929, Mar 2013. [Online]. Available: http://jlt.osa.org/abstract.cfm?URI=jlt-31-6-918
- [29] S. M. LaValle, Planning Algorithms . Cambridge: Cambridge : Cambridge University Press, 2006.
- [30] S. B. Alexander, Optical communication receiver design . IET, 1997, no. 37.
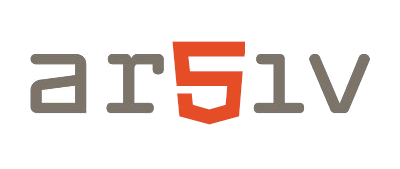
- Help & FAQ
Joint Optimisation of Load Balancing and Handover for Hybrid LiFi and WiFi Networks
- School of Engineering
Research output : Chapter in Book/Report/Conference proceeding › Conference contribution
Abstract / Description of output
Recently a promising concept of hybrid networks based on light fidelity (LiFi) and wireless fidelity (WiFi) emerged. The idea is to combine ultra-small cell LiFi networks with ubiquitous coverage radio frequency (RF) communication systems. In such a hybrid network, WiFi access points (APs) serve a relatively large coverage area with limited bandwidth, and are thus susceptible to traffic overload. This issue is magnified with an increasing number of users because of the inefficient medium access control (MAC) in WiFi systems. LiFi can alleviate this issue by providing additional capacity. LiFi cells, however, have a limited coverage and this could result in significant handover overhead. A conventional load balancing (LB) method optimises the network throughput when the signal-to-noise ratio (SNR) of each user is known and fixed. Although this method delivers maximum throughput at a given time instance, it fails to consider the throughput loss due to handover, especially in an indoor scenario where users may frequently switch between APs. Taking the handover overhead into account, in this paper we propose a novel LB method that focuses on optimising the network throughput over a period of time. Simulation results show that the proposed method can increase the system throughput by up to 70% compared to existing LB methods.
Publication series
Keywords / materials (for non-textual outputs).
- hybrid network
- load balancing
- MOBILE COMMUNICATIONS
Fingerprint
- Wireless Fidelity Computer Science 100%
- Throughput Computer Science 100%
- Load Balancing Computer Science 100%
- Networks Computer Science 100%
- Optimization Computer Science 100%
- User Computer Science 60%
- Hybrid Network Computer Science 40%
- Access Point Computer Science 40%
T1 - Joint Optimisation of Load Balancing and Handover for Hybrid LiFi and WiFi Networks
AU - Wu, Xiping
AU - Safari, Majid
AU - Haas, Harald
N2 - Recently a promising concept of hybrid networks based on light fidelity (LiFi) and wireless fidelity (WiFi) emerged. The idea is to combine ultra-small cell LiFi networks with ubiquitous coverage radio frequency (RF) communication systems. In such a hybrid network, WiFi access points (APs) serve a relatively large coverage area with limited bandwidth, and are thus susceptible to traffic overload. This issue is magnified with an increasing number of users because of the inefficient medium access control (MAC) in WiFi systems. LiFi can alleviate this issue by providing additional capacity. LiFi cells, however, have a limited coverage and this could result in significant handover overhead. A conventional load balancing (LB) method optimises the network throughput when the signal-to-noise ratio (SNR) of each user is known and fixed. Although this method delivers maximum throughput at a given time instance, it fails to consider the throughput loss due to handover, especially in an indoor scenario where users may frequently switch between APs. Taking the handover overhead into account, in this paper we propose a novel LB method that focuses on optimising the network throughput over a period of time. Simulation results show that the proposed method can increase the system throughput by up to 70% compared to existing LB methods.
AB - Recently a promising concept of hybrid networks based on light fidelity (LiFi) and wireless fidelity (WiFi) emerged. The idea is to combine ultra-small cell LiFi networks with ubiquitous coverage radio frequency (RF) communication systems. In such a hybrid network, WiFi access points (APs) serve a relatively large coverage area with limited bandwidth, and are thus susceptible to traffic overload. This issue is magnified with an increasing number of users because of the inefficient medium access control (MAC) in WiFi systems. LiFi can alleviate this issue by providing additional capacity. LiFi cells, however, have a limited coverage and this could result in significant handover overhead. A conventional load balancing (LB) method optimises the network throughput when the signal-to-noise ratio (SNR) of each user is known and fixed. Although this method delivers maximum throughput at a given time instance, it fails to consider the throughput loss due to handover, especially in an indoor scenario where users may frequently switch between APs. Taking the handover overhead into account, in this paper we propose a novel LB method that focuses on optimising the network throughput over a period of time. Simulation results show that the proposed method can increase the system throughput by up to 70% compared to existing LB methods.
KW - attocell
KW - hybrid network
KW - handover
KW - load balancing
KW - MOBILE COMMUNICATIONS
M3 - Conference contribution
T3 - IEEE Wireless Communications and Networking Conference
BT - 2017 IEEE WIRELESS COMMUNICATIONS AND NETWORKING CONFERENCE (WCNC)
PB - Institute of Electrical and Electronics Engineers (IEEE)
T2 - IEEE Wireless Communications and Networking Conference (WCNC)
Y2 - 19 March 2017 through 22 March 2017

IMAGES
COMMENTS
Find & Book the Best Things to Do in Edinburgh. Tours, Activities, Excursions in Edinburgh. Quick & Easy Purchase Process! Full Refund Available up to 24 Hours Before Your Tour Date
Find the Best Things To Do in Edinburgh. Compare Prices and Book Online. Full Refund Available up to 24 Hours Before Your Tour Date. Quick & Easy Purchase Process.
Dr. Majid Safari received PhD degree in Electrical and Computer Engineering from the University of Waterloo, Canada. He is currently a Reader (associate professor) at the Institute for Digital Communication, University of Edinburgh, UK. His research interests include the application of information theory and advanced signal processing in optical wireless, optical fibre, and quantum communications.
Majid SAFARI, Assistant Professor | Cited by 4,304 | of The University of Edinburgh, Edinburgh (UoE) | Read 161 publications | Contact Majid SAFARI
Majid Safari is a Professor of Optical and Wireless Communications at the University of Edinburgh and an associate editor of IEEE Trans. Communications. His main research interest is the application of information theory and signal processing in optical wireless communications.
Professor, University of Edinburgh - 4 819 viittausta - Communication theory - Optical communications - Wireless Communications - Quantum communications ... Majid Safari. Professor, University of Edinburgh. ... MS Islim, S Videv, M Safari, E Xie, JJD McKendry, E Gu, MD Dawson, ... Journal of Lightwave ...
Professor Majid Safari: Institute for Digital Communications Themes, Communications, Signal and Image Processing, Optical Systems and Materials, Sensors, Smart Wireless Devices and Systems +44(0)131 6513569 : [email protected]. Dr Yunjie Yang: Signal and Image Processing, Tomography, Sensors : [email protected]. Dr Kaswar Mostafa
Interested students are asked to contact Prof Majid Safari ([email protected]). Additional application information can be found here. Please note that this vacancy may close at any time, and once a suitable candidate is found. If this role is of interest to you, we recommend you apply as soon as possible.
View Majid Safari's professional profile on LinkedIn. LinkedIn is the world's largest business network, helping professionals like Majid Safari discover inside connections to recommended job candidates, industry experts, and business partners. ... I am delighted to share that I have graduated from the University of Edinburgh with a PhD from ...
Safari, Majid./ MIMO Free-Space Optical Communication.Springer. 2016. pp. 231-253 (Optical Wireless Communications).
Majid Safari's 4 research works with 10 citations and 101 reads, including: Optimal Imaging Receiver Design for High-Speed Mobile Optical Wireless Communications
AU - Safari, Majid. PY - 2020/9/9. Y1 - 2020/9/9. N2 - Free-space optical (FSO) communication provides wireless optical connectivity with high data rates and low-cost implementation; however, its performance is strongly influenced by the power attenuation due to the infrequent adverse weather conditions.
Majid Safari's 3 research works with 2 citations and 41 reads, including: On Optimally Shaped Signals for Nonlinear Frequency Division Multiplexed Fiber Systems
Majid Safari's Post ... France, and University of Edinburgh, UK Title: Hybrid RF/optical links for airborne-underwater data communication Description and how to apply: ...
Shenjie Huang and Majid Safari are with the School of Engineering, the University of Edinburgh, Edinburgh EH9 3JL, U.K. (e-mail: {shenjie.huang, majid.safari}@ed.ac.uk). Cheng Chen and Harald Haas are with LiFi Research and Development
Professor Majid Safari: Institute for Digital Communications Themes, Communications, Signal and Image Processing, Optical Systems and Materials, Sensors, Smart Wireless Devices and Systems +44(0)131 6513569 : [email protected]. Mr Bala Sai Patnam: Dr Steven McDonagh: Signal and Image Processing : [email protected]
M. D. Soltani and M. Safari are with the Institute for Digital Communications (IDCOM), School of Engineering, the University of Edinburgh, Edinburgh, UK. e-mails: fmsoltan, [email protected]. E. Sarbazi, H. Kazemi and H. Haas are with the LiFi Research and Development Centre, Department of Electronic & Electrical
Dr Majid Safari: [email protected] ... The University of Edinburgh is a charitable body, registered in Scotland, with registration number SC005336, VAT Registration Number GB 592 9507 00, and is acknowledged by the UK authorities as a ...
majid safari's 8 research works with 39 citations and 1,127 reads, including: Multi-Hop Quantum Key Distribution with Passive Relays over Underwater Turbulence Channels.
Majid Safari. University of Edinburgh United Kingdom. 1 chapters authored. Related collaborators. Dominique Chiaroni. Ahmad Qidan. Jaafar Elmirghani. King's college London. Mohammad Dehghani Soltani. The University of Edinburgh. Wajahat Ali. The university of Cambridge.
The authors are with the LiFi Research and Development Centre, Institute for Digital Communications, The University of Edinburgh, EH9 3JL, UK. (e-mail: {zhihong.zeng, m.dehghani, cheng.chen, majid.safari, h.haas}@ed.ac.uk). Abstract. Light-fidelity (LiFi) is an emerging technology for high-speed short-range mobile communications. Inter-cell ...
AU - Safari, Majid. AU - Haas, Harald. PY - 2017. Y1 - 2017. N2 - Recently a promising concept of hybrid networks based on light fidelity (LiFi) and wireless fidelity (WiFi) emerged. The idea is to combine ultra-small cell LiFi networks with ubiquitous coverage radio frequency (RF) communication systems.
Majid Safari Professor, University of Edinburgh Verified ... Professor Wasiu O. Popoola School of Engineering, The University of Edinburgh, UK Verified email at ed.ac.uk. Volker Jungnickel Fraunhofer Heinrich ... MD Soltani, L Zhou, M Safari, H Haas. IEEE Communications Surveys & Tutorials 23 (2), 1398-1420, 2021. 196: 2021: Access point ...
Majid Safari Professor, University of Edinburgh Verified email at ed.ac.uk. Aria Nosratinia University of Texas at Dallas Verified email at utdallas.edu. Follow. Mehdi Karbalayghareh. University of Texas at Dallas. Verified email at utdallas.edu. Wireless Communications Information Theory Machine Learning Optical Wireless Communications.